1stGen AHSS, AHSS, Press Hardened Steels, Steel Grades
Introduction
Press hardening steels are typically carbon-manganese-boron alloyed steels. They are also commonly known as:
- Press Hardening Steels (PHS)
- Hot Press Forming Steels (HPF), a term more common in Asia
- Boron Steel: although the name may also refer to other steels, in automotive industry boron steel is typically used for PHS
- Hot Formed Steel (HF), a term more common in Europe.
The most common PHS grade is PHS1500. In Europe, this grade is commonly referred to as 22MnB5 or 1.5528. As received, it has ferritic-pearlitic microstructure and a yield strength between 300-600 MPa depending on the cold working. The tensile strength of as received steel can be expected to be between 450 and 750 MPa. Total elongation must be over a minimum of 12% (A80), but depending on coating type and thickness may well exceed 18% (A80), see Figure 1*. Thus, the grade can be cold formed to relatively complex geometries using certain methods and coatings. When hardened, it has a minimum yield strength of 950 MPa and tensile strength typically around 1300-1650 MPa, Figure 1.B-14 Some companies describe them with their yield and tensile strength levels, such as PHS950Y1500T. It is also common in Europe to see this steel as PHS950Y1300T, and thus aiming for a minimum tensile strength of 1300 MPa after quenching.
The PHS1500 name may also be used for the Zn-coated 20MnB8 or air hardenable 22MnSiB9-5 grades. The former is known as “direct forming with pre-cooling steel” and could be abbreviated as CR1500T-PS, PHS1500PS, PHSPS950Y1300T or similar. The latter grade is known as “multi-step hot forming steel” and could be abbreviated as, CR1500T-MS, PHS1500MS, PHSMS950Y1300T or similar.V-9

Figure 1: Stress-Strain Curves of PHS1500 before and after quenching* (re-created after Citations U-9, O-8, B-18).
In the last decade, several steel makers introduced grades with higher carbon levels, leading to a tensile strength between 1800 MPa and 2000 MPa. Hydrogen induced cracking (HIC) and weldability limit applications of PHS1800, PHS1900 and PHS2000, with studies underway to develop practices which minimize or eliminate these limitations.
Lastly, there are higher energy absorbing, lower strength grades, which have improved ductility and bendability. These fall into two main groups: Press Quenched Steels (PQS) with approximate tensile strength levels of 450 MPa and 550 MPa (noted as PQS450 and PQS550 in Figure 2) and higher ductility PHS grades with approximate tensile strength levels of 1000 and 1200 MPa (shown as PHS1000 and PHS1200 in Figure 2).
Apart from these grades, other grades are suitable for press hardening. Several research groups and steel makers have offered special stainless-steel grades and recently developed Medium-Mn steels for hot stamping purposes. Also, one steel maker in Europe has developed a sandwich material by cladding PHS1500 with thin PQS450 layers on both sides.

Figure 2: Stress-strain curves of several PQS and PHS grades used in automotive industry, after hot stamping for full hardening* (re-created after Citations B-18, L-28, Z-7, Y-12, W-28, F-19, G-30).
PHS Grades with Tensile Strength Approximately 1500 MPa
Hot stamping as we know it today was developed in 1970s in Sweden. The most used steel since then has been 22MnB5 with slight modifications. 22MnB5 means, approximately 0.22 wt-% C, approximately (5/4) = 1.25% wt-% Mn, and B alloying.
The automotive use of this steel started in 1984 with door beams. Until 2001, the automotive use of hot stamped components was limited to door and bumper beams, made from uncoated 22MnB5, in the fully hardened condition. By the end of the 1990s, Type 1 aluminized coating was developed to address scale formation. Since then, 22MnB5 + AlSi coating has been used extensively.B-14
Although some steel makers claim 22MnB5 as a standard material, it is not listed in any international or regional (i.e., European, Asian, or American) standard. Only a similar 20MnB5 is listed in EN 10083-3.T-26, E-3 The acceptable range of chemical composition for 22MnB5 is given in Table 1.S-64, V-9

Table 1: Chemical composition limits for 22MnB5 (listed in wt.%).S-64, V-9
VDA239-500, a draft material recommendation from Verband Der Automotbilindustrie E.V. (VDA), is an attempt to further standardize hot stamping materials. The document has not been published as of early 2021. According to this draft standard, 22MnB5 may be delivered coated or uncoated, hot or cold rolled. Depending on these parameters, as-delivered mechanical properties may differ significantly. Steels for the indirect process, for example, has to have a higher elongation to ensure cold formability.V-9 Figure 1 shows generic stress-strain curves, which may vary significantly depending on the coating and selected press hardening process.
For 22MnB5 to reach its high strength after quenching, it must be austenitized first. During heating, ferrite begins to transform to austenite at “lower transformation temperature” known as Ac1. The temperature at which the ferrite-to-austenite transformation is complete is called “upper transformation temperature,” abbreviated as Ac3. Both Ac1 and Ac3 are dependent on the heating rate and the exact chemical composition of the alloy in question. The upper transformation temperature for 22MnB5 is approximately 835-890 °C.D-21, H-30Austenite transforms to other microstructures as the steel is cooled. The microstructures produced from this transformation depends on the cooling rate, as seen in the continuous-cooling-transformation (CCT) curve in Figure 3. Achieving the “fully hardened” condition in PHS grades requires an almost fully martensitic microstructure. Avoiding transformation to other phases requires cooling rates exceeding a minimum threshold, called the “critical cooling rate,” which for 22MnB5 is 27 °C/s. For energy absorbing applications, there are also tailored parts with “soft zones”. In these soft zones, areas of interest will be intentionally made with other microstructures to ensure higher energy absorption.B-14

Figure 3: Continuous Cooling Transformation (CCT) curve for 22MnB5 (Published in Citation B-19, re-created after Citations M-25, V-10).
Once the parts are hot stamped and quenched over the critical cooling rate, they typically have a yield strength of 950-1200 MPa and an ultimate tensile strength between 1300 and 1700 MPa. Their hardness level is typically between 470 and 510 HV, depending on the testing methods.B-14
Once automotive parts are stamped, they are then joined to the car body in body shop. The fully assembled body known as the Body-in-White (BIW) with doors and closures, is then moved to the paint shop. Once the car is coated and painted, the BIW passes through a furnace to cure the paint. The time and temperature for this operation is called the paint bake cycle. Although the temperature and duration may be different from plant to plant, it is typically close to 170 °C for 20 minutes. Most automotive body components made from cold or hot formed steels and some aluminum grades may experience an increase in their yield strength after paint baking.
In Figure 4, press hardened 22MnB5 is shown in the red curve. In this particular example, the proof strength was found to be approximately 1180 MPa. After processing through the standard 170 °C – 20 minutes bake hardening cycle, the proof strength increases to 1280 MPa (shown in the black curve).B-18 Most studies show a bake hardening increase of 100 MPa or more with press hardened 22MnB5 in industrial conditions.B-18, J-17, C-17

Figure 4: Bake hardening effect on press hardened 22MnB5. BH0 is shown since there is no cold deformation pre-strain. (re-created after Citation B-18).
There are two modified versions of the 22MnB5 recently offered by several steel makers: 20MnB8 and 22MnSiB9-5. Both grades have higher Mn and Si compared to 22MnB5, as shown in Table 2.

Table 2: Chemical compositions of PHS grades with 1500 MPa tensile strength (all listed in wt.%).V-9
Both of these relatively recent grades are designed for Zn-based coatings and are designed for different process routes. For these reasons, many existing hot stamping lines would require some modifications to accommodate these grades.
20MnB8 has been designed for a “direct process with pre-cooling”. The main idea is to solidify the Zn coating before forming, eliminating the possibility that liquid zine fills in the micro-cracks on the formed base metal surface, which in turn eliminates the risk of Liquid Metal Embrittlement (LME). The chemistry is modified such that the phase transformations occur later than 22MnB5. The critical cooling rate of 20MnB8 is approximately 10 °C/s. This allows the part to be transferred from the pre-cooling stage to the forming die. As press hardened, the material has approximately 1000-1050 MPa yield strength and 1500 MPa tensile strength. Once bake hardened (170 °C, 20 minutes), yield strength may exceed 1100 MPa.K-22 This steel may be referred to as PHS950Y1300T-PS (Press Hardening Steel with minimum 950 MPa yield, minimum 1300 MPa tensile strength, for Pre-cooled Stamping).
22MnSiB9-5 has been developed for a transfer press process, named as “multi-step”. As quenched, the material has similar mechanical properties with 22MnB5 (Figure 5). As of 2020, there is at least one automotive part mass produced with this technology and is applied to a compact car in Germany.G-27 Although the critical cooling rate is listed as 5 °C/s, even at a cooling rate of 1 °C/s, hardness over 450HV can be achieved, as shown in Figure 6.H-27 This allows the material to be “air-hardenable” and thus, can handle a transfer press operation (hence the name multi-step) in a servo press. This material is also available with Zn coating.B-15 This steel may be referred to as PHS950Y1300T-MS (Press Hardening Steel with minimum 950 MPa yield, minimum 1300 MPa tensile strength, for Multi-Step process).

Figure 5: Engineering stress-strain curves of 1500 MPa level grades (re-created after Citations B-18, G-29, K-22)

Figure 6: Critical cooling rates of 1500 MPa level press hardening steels (re-created after Citations K-22, H-31, H-27)
Grades with Higher Ductility
Press hardened parts are extremely strong, but cannot absorb much energy. Thus, they are mostly used where intrusion resistance is required. However, newer materials for hot stamping have been developed which have higher elongation (ductility) compared to the most common 22MnB5. These materials can be used in parts where energy absorption is required. These higher energy absorbing, lower strength grades fall into two groups, as shown in Figure 7. Those at the lower strength level are commonly referred to as “Press Quenched Steels” (PQS). The products having higher strength in Figure 7 are press hardening steels since they contain boron and do increase in strength from the quenching operation. The properties listed are after the hot stamping process.
- 450–600 MPa tensile strength level and >15% total elongation, listed as PQS450 and PQS550.
- 1000–1300 MPa tensile strength level and >5% total elongation, listed as PHS1000 and PHS1200.

Figure 7: Stress-strain curves of several PQS and PHS grades used in automotive industry, after hot stamping for full hardening* (re-created after Citations B-18, Y-12).
Currently none of these grades are standardized. Most steel producers have their own nomination and standard, as summarized in Table 3. There is a working document by German Association of Automotive Industry (Verband der Automobilindustrie, VDA), which only specifies one of the PQS grades. In the draft standard, VDA239-500, PQS450 is listed as CR500T-LA (Cold Rolled, 500 MPa Tensile strength, Low Alloyed). Similarly, PQS550 is listed as CR600T-LA.V-9 Some OEMs may prefer to name these grades with respect to their yield and tensile strength together, as listed in Table 3.
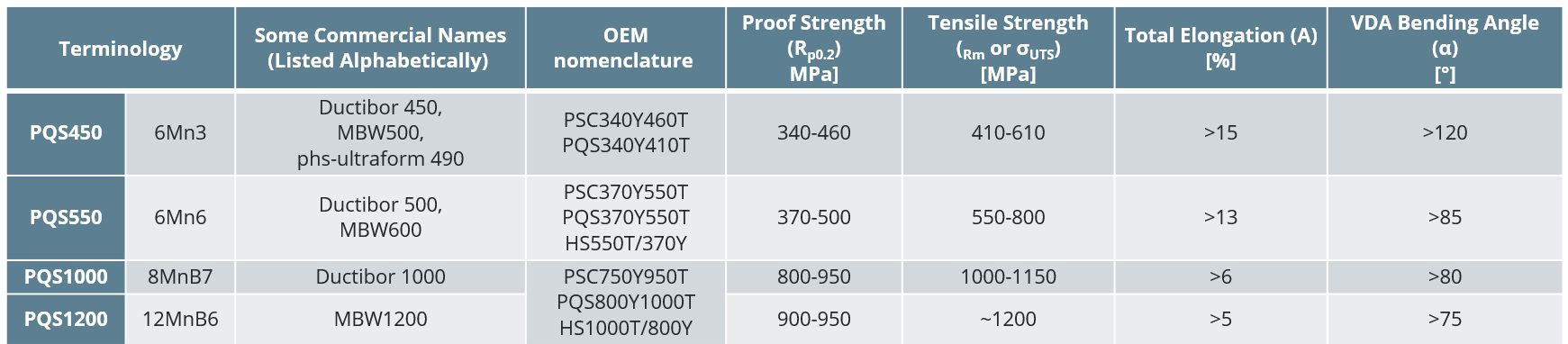
Table 3: Summary of Higher Ductility grades. The terminology descriptions are not standardized. Higher Ductility grade names are based on their properties and terminology is derived from a possible chemistry or OEM description. The properties listed here encompass those presented in multiple sources and may or may not be associated with any one specific commercial grade.Y-12, T-28, G-32
PQS grades have been under development at least since 2002. In the earliest studies, PQS 1200 was planned.R-11 Between 2007 and 2009, three new cars were introduced in Europe, having improved “energy absorbing” capacity in their hot stamped components. VW Tiguan (2007-2016) and Audi A5 Sportback (2009-2016) had soft zones in their B-pillars (Figure 8B and C). Intentionally reducing the cooling rate in these soft zone areas produces microstructures having higher elongations. In the Audi A4 (2008-2016) a total of three laser welded tailored blanks were hot stamped. The soft areas of the A4 B-pillars were made of HX340LAD+AS (HSLA steel, with AlSi coating, as delivered, min yield strength = 340 MPa, tensile strength = 410-510 MPa) as shown in Figure 8A. After the hot stamping process, HX340LAD likely had a tensile strength between 490 and 560 MPaS-65, H-32, B-20, D-22, putting it in the range of PQS450 (see Table 3). Note that there were not the only cars to have tailored hot stamped components during that time.
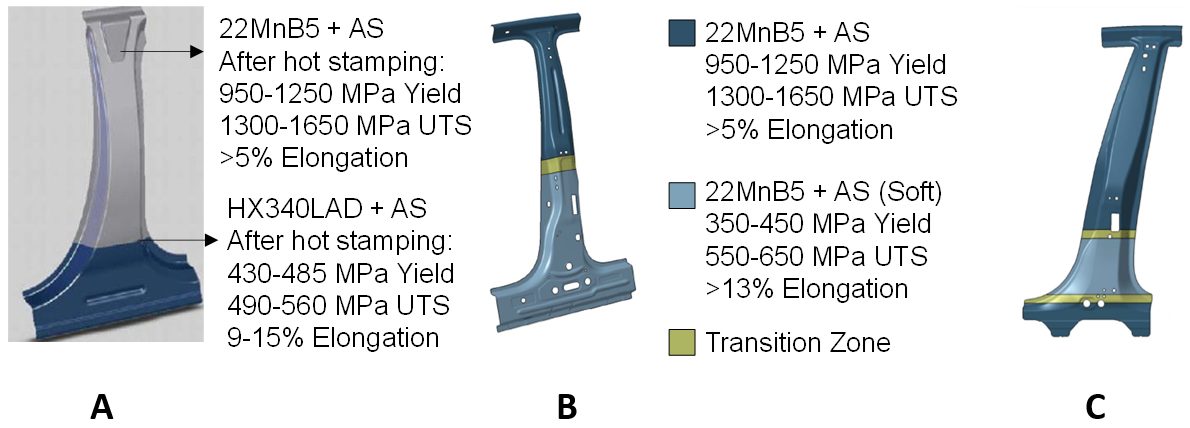
Figure 8: Earliest energy absorbing hot stamped B-pillars: (A) Audi A4 (2008-2016) had a laser welded tailored blank with HSLA material; (B) VW Tiguan (2007-2015) and (C) Audi A5 Sportback (2009-2016) had soft zones in their B-pillars (re-created after Citations H-32, B-20, D-22).
A 2012 studyK-25 showed that a laser welded tailored B-Pillar with 340 MPa yield strength HSLA and 22MnB5 had the best energy absorbing capacity in drop tower tests, compared to a tailored (part with a ductile soft-zone) or a monolithic part, Figure 9. As HSLA is not designed for hot stamping, most HSLA grades may have very high scatter in the final properties after hot stamping depending on the local cooling rate. Although the overall part may be cooled at an average 40 to 60 °C/s, at local spots the cooling rate may be over 80 °C/s. PQS grades are developed to have stable mechanical properties after a conventional hot stamping process, in which high local cooling rates may be possible.M-26, G-31, T-27

Figure 9: Energy absorbing capacity of B-pillars increase significantly with soft zones or laser welded tailored blank with ductile material (re-created after Citation K-25).
PQS grades have been in use at latest since 2014. One of the earliest cars to announce using PQS450 was VolvoXC90. There are six components (three right + three left), tailor welded blanks with PQS450, as shown Figure 10.L-29 Since then, many carmakers started to use PQS450 or PQS550 in their car bodies. These include:
- Fiat 500X: Patchwork supported, laser welded tailored rear side member with PQS450 in crush zonesD-23,
- Fiat Tipo (Hatchback and Station Wagon versions): similar rear side member with PQS450B-14,
- Renault Scenic 3: laser welded tailored B-pillar with PQS550 in the lower sectionF-19,
- Chrysler Pacifica: five-piece front door ring with PQS550 in the lower section of the B-Pillar areaT-29, and
- Chrysler Ram: six-piece front door ring with PQS550 in the lower section of the B-Pillar area.R-3
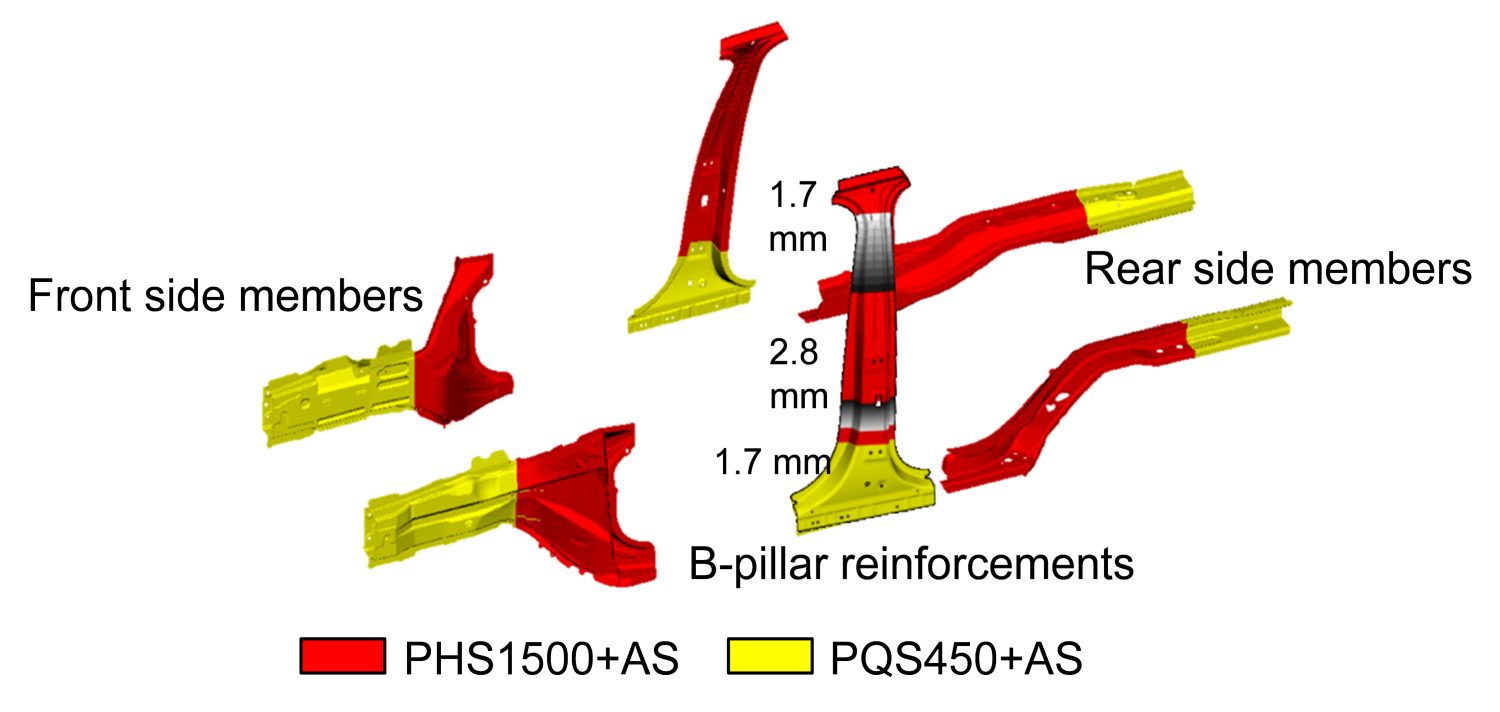
Figure 10: Use of laser welded tailored PQS-PHS grades in 2nd generation Volvo XC90 (re-created after Citation L-29).
Several car makers use PQS grades to facilitate joining of components. The B-Pillar of the Jaguar I-PACE electric SUV is made of PQS450, with a PHS1500 patch that is spot welded before hot stamping, creating the patchwork blank shown in Figure 11A.B-21 Early PQS applications involved a laser welded tailored blank with PHS 1500. Since 2014, Mercedes hot stamped PQS550 blanks not combined with PHS1500. Figure 11B shows such components on the Mercedes C-Class.K-26
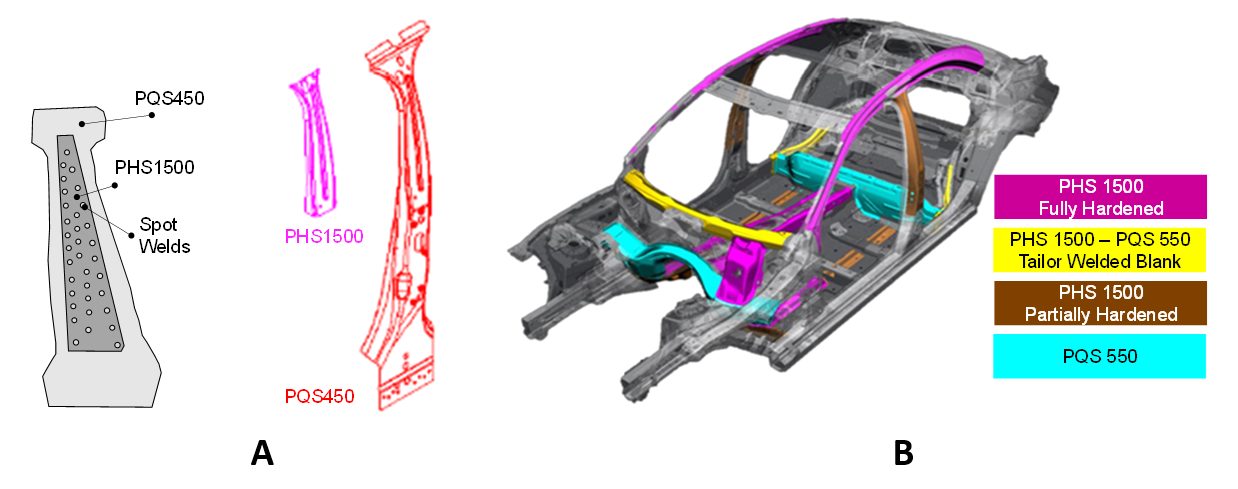
Figure 11: Recent PQS applications: (a) 2018 Jaguar I-PACE uses a patchwork B-pillar with PQS450 master blank and PHS1500 patchB-21, (b) 2014 Mercedes C-Class has a number of PQS550 components that are not laser welded to PHS1500.K-26
PHS Grades over 1500 MPa
The most commonly used press hardening steels have 1500 MPa tensile strength, but are not the only optionsR-11, with 4 levels between 1700 and 2000 MPa tensile strength available or in development as shown in Figure 12. Hydrogen induced cracking (HIC) and weldability problems limit widespread use in automotive applications, with studies underway to develop practices which minimize or eliminate these limitations.
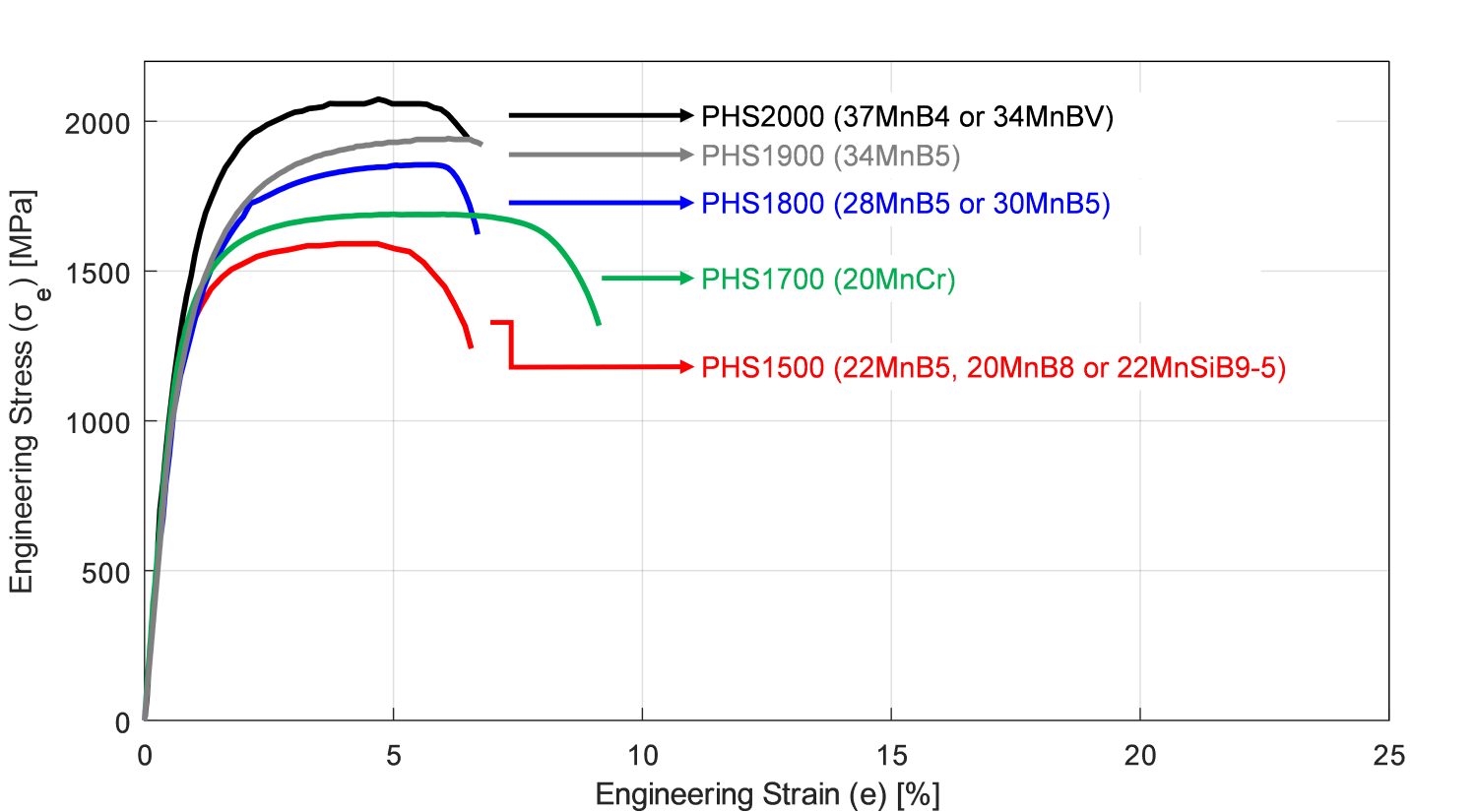
Figure 12: PHS grades over 1500 MPa tensile strength, compared with the common PHS1500 (re-created after Citations B-18, W-28, Z-7, L-30, L-28, B-14).
Mazda Motor Corporation was the first vehicle manufacturer to use higher strength boron steels, with the 2011 CX-5 using 1,800MPa tensile strength reinforcements in front and rear bumpers, Figure 13. According to Mazda, the new material saved 4.8 kg per vehicle. The chemistry of the steel is Nb modified 30MnB5.H-33, M-28 Figure 14 shows the comparison of bumper beams with PHS1500 and PHS1800. With the higher strength material, it was possible to save 12.5% weight with equal performance.H-33

Figure 13: Bumper beam reinforcements of Mazda CX-5 (SOP 2011) are the first automotive applications of higher strength boron steels.M-28

Figure 14: Performance comparison of bumper beams with PHS1500 and PHS1800.H-33
PHS 1800 is used in the 2022 Genesis Electrified G80 (G80EV) and the new G90, both from Hyundai Motor. A specialized method lowering the heating furnace temperature by more than 50℃ limits the penetration of hydrogen into the blanks, minimizing the risk of hydrogen embrittlement. L-64.
MBW 1900 is the commercial name for a press hardening steel with 1900 MPa tensile strength. An MBW 1900 B-pillar with correct properties can save 22% weight compared to DP 600 and yet may cost 9% less than the original Dual-Phase design.H-34 Ford had also demonstrated that by using MBW 1900 instead of PHS 1500, a further 15% weight could be saved.L-30 Since 2019, VW’s electric vehicle ID.3 has two seat crossbeams made of MBW 1900 steel, as seen in Figure 15.L-31 The components are part of MEB platform (Modularer E-Antriebs-Baukasten – modular electric-drive toolkit) and may be used in other VW Group EVs.
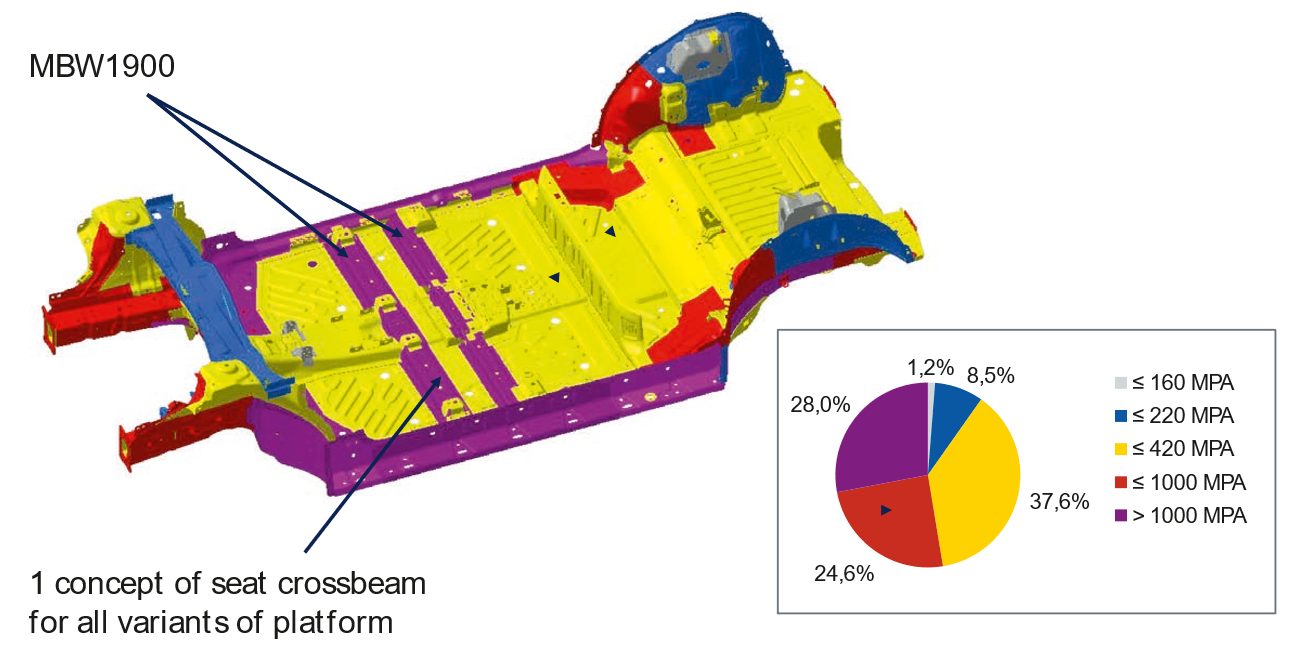
Figure 15: Underbody of VW ID3 (part of MEB platform).L-31
USIBOR 2000 is the commercial name given to a steel grade similar to 37MnB4 with an AlSi coating. Final properties are expected only after paint baking cycle, and the parts made with this grade may be brittle before paint bake.B-32 In June 2020, Chinese Great Wall Motors started using USIBOR 2000 in the Haval H6 SUV.V-12
HPF 2000, another commercial name, is used in a number of component-based examples, and also in the Renault EOLAB concept car.L-28, R-12 An 1800 MPa grade is under development.P-22 Docol PHS 1800, a commercial grade approximating 30MnB5, has been in production, with Docol PHS 2000 in development.S-66 PHS-Ultraform 2000, a commercial name for a Zn (GI) coated blank, is suited for the indirect process.V-11
General Motors China, together with several still mills across the country, have developed two new PHS grades: PHS 1700 (20MnCr) and PHS2000 (34MnBV). 20MnCr uses Cr alloying to improve hardenability and oxidation resistance. This grade can be hot formed without a coating. The furnace has to be conditioned with N2 gas. The final part has high corrosion resistance, approximately 9% total elongation (see Figure 12) and high bendability (see Table 4). 34MnBV on the other hand, has a thin AlSi coating (20g/m2 on each side). Compared with the typical thickness of AlSi coatings, thinner coatings are preferred for bendability (see Table 5).W-28 More information about these oxidation resistant PHS grades, as well as a 1200 MPa version intended for applications benefiting from enhanced crash energy absorption, can be found in Citation L-60.
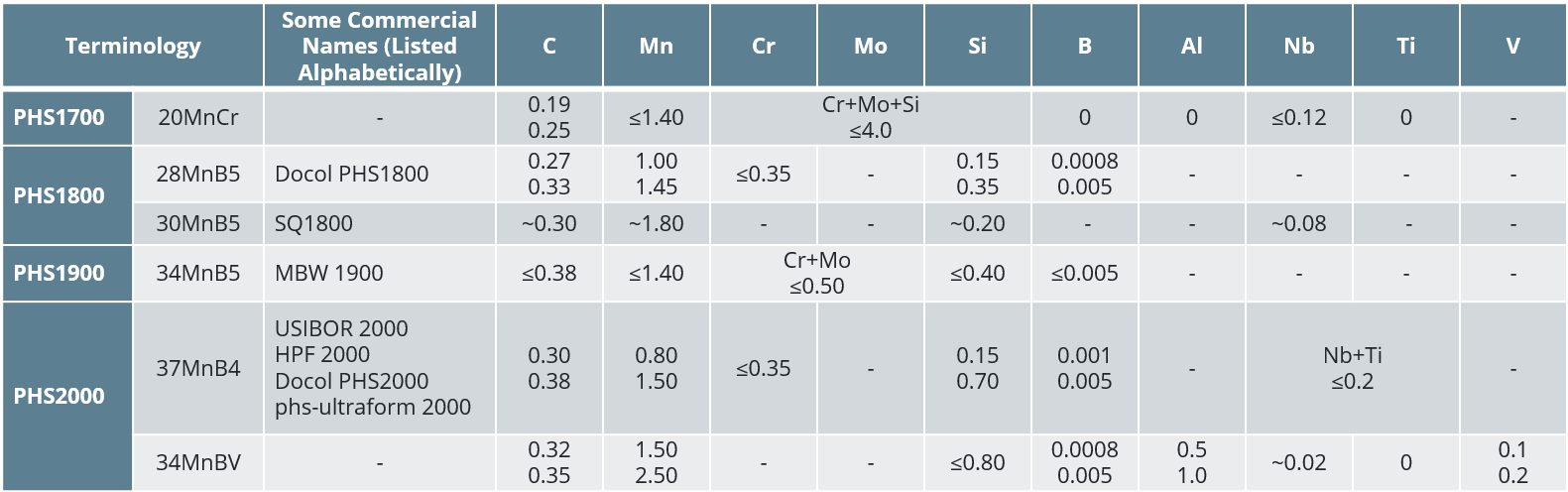
Table 4: Chemical compositions of higher strength PHS grades. “0” means it is known that there is no alloying element, while “-” means there is no information. “~” is used for typical values; otherwise, minimum or maximum are given. The terminology descriptions are not standardized. PQS names are based on their properties and grade names are derived from a possible chemistry or OEM description. The properties listed here encompass those presented in multiple sources and may or may not be associated with any one specific commercial grade.W-28, B-32, H-33, G-33, L-28, S-67, S-66, Y-12, B-33
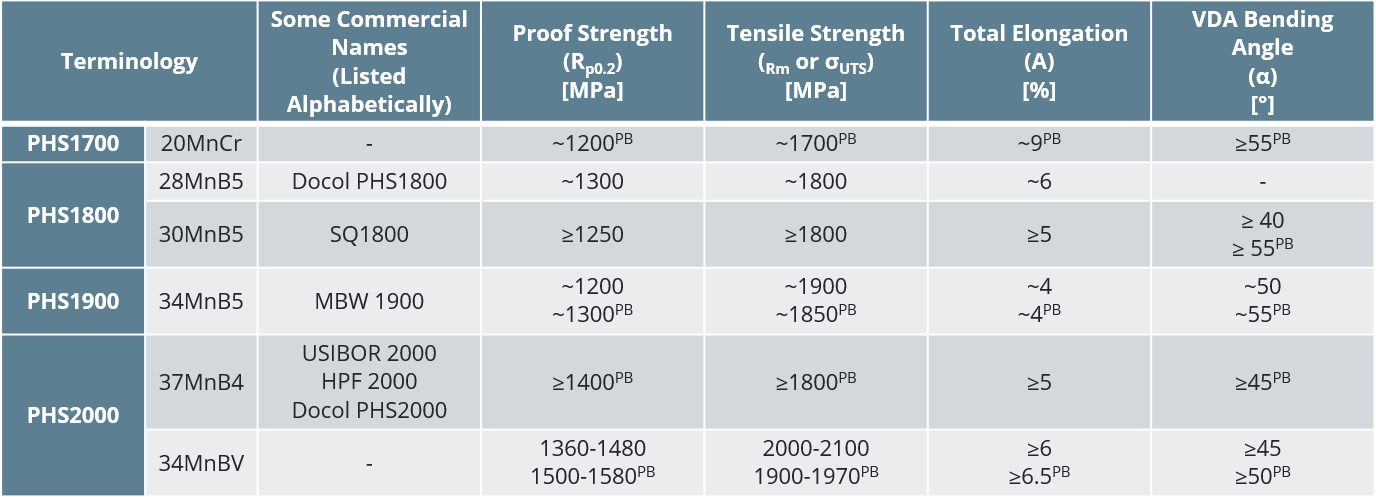
Table 5: Mechanical properties of higher strength PHS grades. “~” is used for typical values; otherwise, minimum or maximum are given. Superscript PB means after paint bake cycle. The terminology descriptions are not standardized. PQS names are based on their properties and grade names are derived from a possible chemistry or OEM description. The properties listed here encompass those presented in multiple sources and may or may not be associated with any one specific commercial grade.W-28, B-32, H-33, G-33, L-28, S-67, S-66, Y-12
Other Steels for Press Hardening Process
In recent years, many new steel grades are under evaluation for use with the press hardening process. Few, if any, have reached mass production, and are instead in the research and development phase. These grades include:
- Stainless steels
- Medium-Mn steels
- Composite steels
Stainless Steels
Studies of press hardening of stainless steels primarily focus on martensitic grades (i.e., AISI SS400 series).M-36, H-42, B-40, M-37, F-30 As seen in Figure 16, martensitic stainless steels may have higher formability at elevated temperatures, compared to PHS1500 (22MnB5). Other advantages of stainless steels are:
- better corrosion resistanceM-37,
- potentially higher heating rates (i.e., induction heating) F-30,
- possibility of air hardening – allowing the multi-step process — as seen in Figure 17a H-42,
- high cold formability – allowing indirect process – as seen in Figure 17b.M-37
Disadvantages include (a) higher material cost, and (b) higher furnace temperature (up to around 1050-1150 °C).M-37, F-30 As of 2020, there are two commercially available stainless steel grades specifically developed for press hardening process.

Figure 16: Tensile strength and total elongation variation with temperature of (a) PHS1500 = 22MnB5M-38 and (b) martensitic stainless steel.M-36

Figure 17: (a) Critical cooling rate comparison of 22MnB5 and AISI SS410 (re-created after Citation H-42), (b) Room temperature forming limit curve comparison of DP600 and modified AISI SS410 (re-created after Citation M-37).
Final mechanical properties of stainless steels after press hardening process are typically superior to 22MnB5, in terms of elongation and energy absorbing capacity. Figure 18 illustrates engineering stress-strain curves of the commercially available grades (1.6065 and 1.4064), and compares them with the 22MnB5 and a duplex stainless steel (Austenite + Martensite after press hardening). These grades may also have bake hardening effect, abbreviated as BH0, as there will be no cold deformation.B-40, M-37, F-30

Figure 18: Engineering Stress-Strain curves of press hardened stainless steels, compared with 22MnB5 (re-created after Citations B-40, M-37, F-30, B-41).

Table 6: Summary of mechanical properties of press hardenable stainless steel grades. Typical values are indicated with “~”. (Table generated from Citations B-40, M-37, F-30.)
Medium-Mn Steels
Medium-Mn steels typically contain 3 to 12 weight-% manganese alloying.D-27, H-30, S-80, R-16, K-35 Although these steels were originally designed for cold stamping applications, there are numerous studies related to using them in the press hardening process as well.H-30 Several advantages of medium-Mn steels in press hardening are:
- Austenitization temperature may be significantly lower than compared to 22MnB5, as indicated in Figure 19.H-30, S-80 Thus, using medium-Mn steels may save energy in heating process.M-39 Lower heating temperature may also help reducing the liquid-metal embrittlement risk of Zn-coated blanks. It also may reduce oxidation and decarburization of uncoated blanks.S-80
- Martensitic transformation can occur at low cooling rates. Simpler dies could be used with less or no cooling channels. In some grades, air hardening may be possible. Thus, multi-step process could be employed.S-80, B-14
- Some retained austenite may be present at the final part, which can enhance the elongation, through the TRIP effect. This, in turn, improves toughness significantly.S-80, B-14

Figure 19: Effect of Mn content on equilibrium transformation temperatures (re-created after Citations H-30, B-14)
The change in transformation temperatures with Mn-alloying was calculated using ThermoCalc software.H-30 As seen in Figure 19, as Mn alloying is increased, austenitization temperatures are lowered.H-30 For typical 22MnB5 stamping containing 1.1 to 1.5 % Mn, furnace temperature is typically set at 930 °C in mass production. The multi-step material 22MnSiB9-5 has slightly higher Mn levels (2.0 to 2.4 %), so the furnace temperature could be reduced to 890 °C. As also indicated in Table 7, the furnace temperature could be further lowered in hot forming of medium-Mn steels.
A study in the EU showed that if the maximum furnace temperature is 930 °C, which is common for 22MnB5, natural gas consumption will be around 32 m3/hr. In the study, two new medium-Mn steels were developed, one with 3 wt.% Mn and the other with 5 wt% Mn. These grades had lower austenitization temperature, and the maximum furnace set temperature could be reduced to 808 °C and 785 °C, respectively. Experimental data shows that at 808 °C natural gas consumption was reduced to 19 m3/hr, and at 785 °C to 17 m3/hr.M-39 In Figure 20, experimental data is plotted with a curve fit. Based on this model, it was estimated that by using 22MnSiB9-5, furnace gas consumption may be reduced by 15%.

Figure 20: Effect of maximum furnace set temperature (at the highest temperature furnace zone) on natural gas consumption (raw data from Citation M-39)
Lower heating temperature of medium-Mn steels may also help reducing the liquid-metal embrittlement risk of Zn-coated blanks. It also may reduce oxidation and decarburization of uncoated blanks.S-80
Medium-Mn steels may have high yield-point elongation (YPE), with reports of more than 5% after hot stamping. Mechanical properties may be sensitive to small changes in temperature profile. As seen in Figure 21, all studies with medium-Mn steel have a unique stress-strain curve after press hardening. This can be explained by:
- differences in the chemistry,
- thermomechanical history of the sheet prior to hot stamping,
- heating rate, heating temperature and soaking time, and
- cooling rate.S-80

Figure 21: Engineering Stress-Strain curves of several press hardened medium-Mn steels, compared with 22MnB5. See Table 7 for an explanation of each tested material (re-created after Citations S-80,L-37, W-30, L-38).

Table 7: Summary of mechanical properties of press hardenable Medium-Mn grades shown in Figure 18. Typical values are indicated with “~”. Toughness is calculated as the area under the engineering stress-strain curve. Items 4 and 5 also were annealed at different temperatures and therefore have different thermomechanical history. Note that these grades are not commercially available.L-38, W-30, L-37, S-80
Composite Steels
TriBond ® is the name given to a family of steel composites.T-32 Here, three slabs (one core material (60 to 80% of the thickness) and two cladding layers) are surface prepared, stacked on top of each other, and welded around the edges. The stack is hot rolled to thickness. Cold rolling could also be applied. Initially, TriBond ® was designed for wear-resistant cladding and ductile core materials.
The original design was optimized for hot stamping.B-14 The core material, where bending strains are lower than the outer layers, is made from generic 22MnB5 (PHS1500). Outer layers are made with PQS450. The stack is cold rolled, annealed and AlSi coated.Z-9 Two grades are developed, differing by the thickness distribution between the layers, as shown in Figure 22.R-14

Figure 22: Sample microsections of the conventional hot stamping grade PHS1500+AS, the high strength composite Tribond® 1400 and the high energy absorbing composite Tribond® 1200. The Tribond® 1200 microsection is experimental and is taken from Citation R-14. The other two images are renditions created by the author for explanation purposes. (re-created after Citations R-14, R-15)
Total elongation of the composite steel is not improved, compared to PHS1500, as shown in Figure 23. The main advantage of the composite steels is their higher bendability, as seen in Table 8. Crashboxes, front and rear rails, seat crossmembers and similar components experience axial crush loading in the event of a crash. In axial crush, Tribond® 1200 saved 15% weight compared to DP780 (CR440Y780T-DP). The bending loading mode effects B-pillars, bumper beams, rocker (sill) reinforcements, side impact door beams, and similar components during a crash. In this bending mode, Tribond® 1400 saved 8 to 10% weight compared to regular PHS1500. Lightweighting cost with Tribond® 1400 was calculated as €1.50/kgsaved.G-37, P-26

Figure 23: Engineering Stress-Strain curves of core layer, outer layer and the composite steel (re-created after Citation P-26).

Table 8: Summary of composite steels and comparison with conventional PHS and PQS grades. Typical values are indicated with “~”. (Table re-created after Citation B-14).
* Graphs in this article are for information purposes only. Production materials may have different curves. Consult the Certified Mill Test Report and/or characterize your current material with an appropriate test (such as a tensile, bending, hole expansion, or crash test) test to get the material data pertaining to your current stock.
For more information on Press Hardened Steels, see these pages:
Back To Top
Metallurgy
Basis
There are different ways to classify automotive steels. One is a metallurgical designation providing some process information. Common designations include lower-strength steels (interstitial-free and mild steels); conventional high strength steels, such as bake hardenable and high-strength, low-alloy steels (HSLA); and Advanced High-Strength Steels (AHSS) such as dual phase and transformation-induced plasticity steels. Additional higher strength steels include press hardening steels and steels designed for unique applications that have improved edge stretch and stretch bending characteristics.
A second classification method important to part designers is strength of the steel. This document will use the general terms HSLA and AHSS to designate all higher strength steels. The principal difference between conventional HSLA steels and AHSS is their microstructure. Conventional HSLA steels are single-phase ferritic steels with a potential for some pearlite in C-Mn steels. AHSS are primarily steels with a multiphase microstructure containing one or more phases other than ferrite, pearlite, or cementite – for example martensite, bainite, austenite, and/or retained austenite in quantities sufficient to produce unique mechanical properties. Some types of AHSS have a higher strain hardening capacity resulting in a strength-ductility balance superior to conventional steels. Other types have ultra-high yield and tensile strengths and show a bake hardening behavior.
AHSS include all martensitic and multiphase steels having a minimum specified tensile strength of at least 440 MPa. Those steels with very high minimum specified tensile strength are sometimes referred to as Ultra High Strength Steels (UHSS). Several companies choose 980 MPa as the threshold where “Ultra” high strength begins, while others use higher thresholds of 1180 MPa or 1270 MPa. There is no generally accepted definition among the producers or users of the product. The difference between AHSS and UHSS is in terminology only – they are not separate products. The actions taken by the manufacturing community to form, join, or process is ultimately a function of the steel grade, thickness, and mechanical properties. Whether these steels are called “Advanced” or “Ultra” does not impact the technical response.
Third Generation, or 3rd Gen, AHSS builds on the previously developed 1st Gen AHSS (DP, TRIP, CP, MS, and PHS) and 2nd Gen AHSS (TWIP), with global commercialization starting around 2020. 3rd Gen AHSS are multi-phase steels engineered to develop enhanced formability as measured in tensile, sheared edge, and/or bending tests. Typically, these steels rely on retained austenite in a bainite or martensite matrix and potentially some amount of ferrite and/or precipitates, all in specific proportions and distributions, to develop these enhanced properties.
Nomenclature
Historically, HSLA steels were described by their minimum yield strength. Depending on the region, the units may have been ksi or MPa, meaning that HSLA 50 and HSLA 340 both describe a High Strength Low Alloy steel with a minimum yield strength of 50 ksi = 50,000 psi ≈ 340 MPa. Although not possible to tell from this syntax, many of the specifications stated that the minimum tensile strength was 70 MPa to 80 MPa greater than the minimum yield strength.
Development of the initial AHSS grades evolved such that they were described by their metallurgical approach and minimum tensile strength, such as using DP590 to describe a dual phase steel with 590 MPa tensile strength. Furthermore, when Advanced High Strength Steels were first commercialized, there was often only one option for a given metallurgical type and tensile strength level. Now, for example, there are multiple distinct dual phase grades with a minimum 980 MPa tensile strength, each with different yield strength or formability.
To highlight these different characteristics throughout this website, each steel grade is identified by whether it is hot rolled or cold rolled, minimum yield strength (in MPa), minimum tensile strength (in MPa), and metallurgical type. Table 1 lists different types of steels.

Table 1: Different Types of Steels and Associated Abbreviations.
As an example, CR-500Y780T-DP describes a cold rolled dual phase steel with 500 MPa minimum yield strength and 780 MPa minimum ultimate tensile strength. There is also another grade with the same minimum UTS, but lower yield strength: CR440Y780T-DP. If the syntax is simply DP780, the reader should assume either that the referenced study did not distinguish between the variants or that the issues described in that section applies to all variants of a dual phase steel with a minimum 780 MPa tensile strength.
Another syntax issue is the presentation of the strength (yield or tensile), and whether it is rounded to the nearest 10 or 50 MPa. For example, consider DP980 compared with DP1000. Both forms represent essentially the same grade. In Europe, this steel may be described as having a tensile strength of 100 kgf/mm2, corresponding to 981 N/mm2 (981 MPa), and expressed as DP980. In Asia, the steel may be referred to as 100K (an abbreviation for 100 kgf/mm2). In other parts of the world, it may be rounded to nearest 50 MPa, as DP 1000. This naming approach applies to many grades, with some shown in Table 2. In some cases, although the OEM specification may list the steel as DP800 (for example), the minimum tensile strength requirement may still be 780 MPa. Furthermore, independent of the chosen naming syntax, the steel company will supply to the actual specification requirements, and will use different process controls to meet a 780 MPa minimum compared with an 800 MPa minimum.
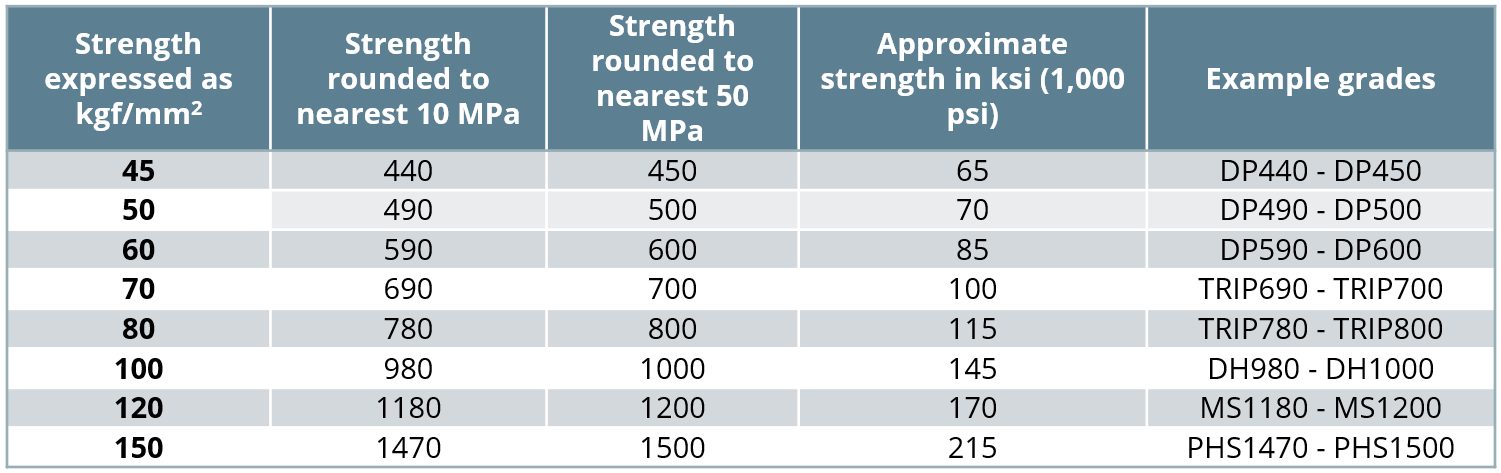
Table 2: Syntax Related to AHSS Strength Levels
Press hardening steels sometimes require a different syntax. Some OEMs will use a similar terminology as described above. For example: CR-950Y1300T-PH (PH stands for Press Hardenable or Press Hardened) or CR-950Y1300T-MB (MB stands for Manganese-Boron steel) can describe the same cold rolled press hardening steel with 950 MPa minimum yield strength and 1300 MPa minimum tensile strength after completing the press hardening operation. Other specifications may show suffixes which highlight the forming process used, such as -DS for direct hot stamping, -IS for indirect hot stamping and MS for a multi-step process. Furthermore, sources may describe this product focused on its typical tensile strength as PHS1500T. The abbreviation PQS (Press Quenched Steel) is typically used for grades that do not harden after hot stamping. These may be noted as PQS450 and PQS550, where the numbers stand for the approximate minimum tensile strength after the hot stamping cycle (see the section on Grades With Higher Ductility on the linked page).
Graphical Presentation
Generally, elongation (a measure of ductility) decreases as strength increases. Plotting elongation on the vertical axis and strength on the horizontal axis leads to a graph starting in the upper left (high elongation, lower strength) and progressing to the lower right (lower elongation, higher strength). This shape led to the colloquial description of calling this the banana diagram.

Figure 1: A generic “banana” diagram comparing strength and elongation.
With the continued development of advanced steel options, it is no longer appropriate to describe the plethora of options as being in the shape of a banana. Instead, with new grades filling the upper right portion (see Figure 2), perhaps it is more accurate to describe this as the football diagram as the options now start to fall into the shape of an American or Rugby Football. Officially, it is known as the steel Global Formability Diagram.
Even this approach has its limitations. Elongation is only one measure of ductility. Other ductility parameters are increasingly important with AHSS grades, such as hole expansion and bendability. BillurB-61 proposed a diagram comparing the bend angle determined from the VDA238-100 testV-4 with the yield strength for various press hardened and press quenched steels.

Figure 3: VDA Bending Angle typically decreases with increasing yield strength of PHS/PQS grades.B-61
Figure 4 shows a local/global formability map sometimes referred to as the Hance Diagram named after the researcher who proposed it.H-16 This diagram combines measures of local formability (characterized by true fracture strain) and global formability (characterized by uniform elongation), providing insight on different characteristics associated with many steel grades and helping with application-specific material grade selection. For example, if good trim conditions still create edge splits, selecting materials higher on the vertical axis may help address the edge-cracking problems. Likewise, global formability necking or splitting issues can be solved by using grades further to the right on the horizontal axis.

Figure 4: The Local/Global Formability Map combines measures of local formability (true fracture strain) and global formability (uniform elongation) to highlight the relative characteristics of different grades. In this version from Citation D-12, the colors distinguish different options at each tensile strength level.
Grade Portfolio
Previous AHSS Application Guidelines showcased a materials portfolio driven by the FutureSteelVehicle (FSV) program, with more than twenty new grades of AHSS acknowledged as commercially available by 2020. The AHSS materials portfolio continues to grow, as the steel industry responds to requirements for high strength, lightweight steels. Table 3 reflects available AHSS grades as well as grades under development and nearing commercial application. The Steel Grades page provides details about these grades and their applications.

Table 3: Commercially available AHSS Grades and grades under development for near-term application. Grade names shown in Italicized Bold were available in FutureSteelVehicle. For all but PQS/PHS, Grade Name indicates the minimum yield strength, minimum tensile strength, and the type of AHSS. The min EL column indicates a typical minimum total elongation value, which may vary based on test sample shape, gauge length, and thickness. PQS/PHS grade name indicates nominal tensile strength.
Global automakers create steel specification criteria suited for their vehicle targets, manufacturing infrastructure, and other constraints. Although similar specifications exist at other companies, perfect overlap of all specifications is unlikely. Global steelmakers have different equipment, production capabilities, and commercial availability.
Minimum or typical mechanical properties shown on this web page and throughout this site illustrates the broad range of AHSS grades that may be available. Properties of hot rolled steels can differ from cold rolled steels. Coating processes like hot dip galvanizing or galvannealing subjects the base metal to different thermal cycles that affect final properties. Test procedures and requirements have a regional or OEM influence, such as preference to using tensile test gauge length of 50 mm or 80 mm, or specifying minimum property values parallel or perpendicular to the rolling direction.
Steel users must communicate directly with individual steel companies to determine specific grade availability and the specific associated parameters and properties, such as:
- Chemical composition specifications,
- Mechanical properties and ranges,
- Thickness and width capabilities,
- Hot-rolled, cold-rolled, and coating availability,
- Joining characteristics.