1stGen AHSS, 2ndGen AHSS, 3rdGen AHSS, AHSS, Blog, homepage-featured-top, main-blog, News, Production Managers, Steel Grades, Tool & Die Professionals
This month’s blog was contributed by Peter Ulintz, Precision Metalforming Association. This content originally appeared in the September 2023 issue of MetalForming Magazine under the title “Stronger AHSS Knowledge Required for Metal Stampers” and has been reproduced with the permission of MetalForming Magazine.
Metal stampers and die shops experienced with mild and HSLA steels often have problems making parts from AHSS grades. The higher initial yield strengths and increased work hardening of these steels can require as much as four times the working loads of mild steel. Some AHSS grades also have hardness levels approaching the dies used to form them.
Dies Get Tougher
Metal stampers and die shops experienced with mild and HSLA steels often have problems making parts from AHSS grades. The higher initial yield strengths and increased work hardening of these steels can require as much as four times the working loads of mild steel. Some AHSS grades also have hardness levels approaching the dies used to form them.
The higher stresses required to penetrate higher-strength materials require increased punch-to-die clearances compared to mild steels and HSLA grades. Why? This clearance acts as leverage to bend and break the slug out of the sheet metal. Stronger materials need longer levers to bend the slug. The required clearance is a function of the steel grade and tensile strength, and sheet thickness.
Increasing cutting clearance can result in punch cracking and head breakage due to higher snapthrough loads and reverse-unloading forces within the die. Adding shear angles to the punch face helps reduce punch forces and reverse unloading.
Tight-cutting clearances increase the tendency for die galling and chipping. The severity of galling depends on the surface finish and microstructure of both the tool steel and work material. Chipping can occur when process stresses are high enough to cause low-cycle fatigue of the tooling material, indicating that the material lacks toughness.
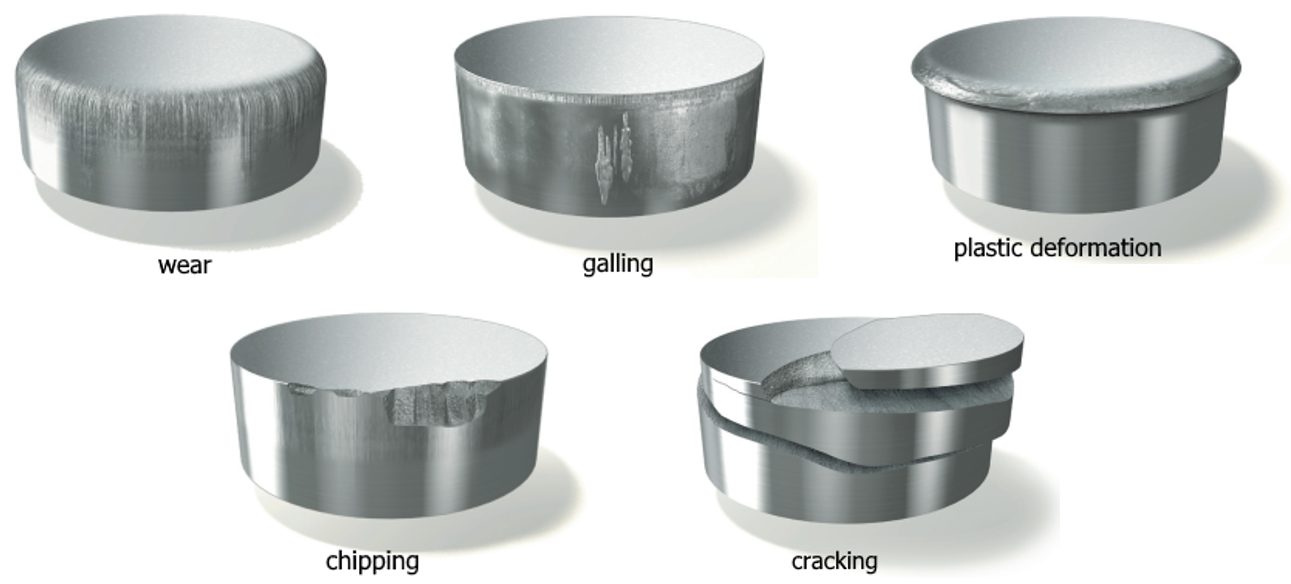
Stamping Tool Failure Modes (Citations T-20 and U-7)
Tempering of tools and dies represents a critical heat-treatment step and serves more than one purpose, but of primary concern is the need to relieve residual stresses and impart toughness. Dies placed in service without proper tempering likely will experience early failure.
Dies made from the higher-alloy tool-steel grades (D, M or T grades) require more than one tempering step. These grades contain large amounts of retained austenite and untempered martensite after the first tempering step and require at least one more temper to relieve internal stresses, and sometimes a third temper for even greater toughness.
Unfortunately, heat treatment remains a “black-box” process for most die shops and manufacturing companies, which send soft die details to the local heat treat facility, with hardened details returned. A cursory Rockwell hardness test may be conducted at the die shop when the parts return. If they meet hardness requirements, the parts usually are accepted, regardless of how they may have been processed—a problem, as hardness alone does not adequately measure impact toughness.
Machines Get Stronger
The increased forces needed to form, cut and trim higher-strength steels create significant challenges for pressroom equipment and tooling. These include excessive tooling deflections, damaging tipping-moments, and amplified vibrations and snapthrough forces that can shock and break dies—and sometimes presses. Stamping AHSS materials can affect the size, strength, power and overall configuration of every major piece of the press line, including material-handling equipment, coil straighteners, feed systems and presses.
Here is what every stamper should know about higher-strength materials:
- Because higher-strength steels require more stress to deform, additional servo motor power and torque capability may be needed to pull the coil material through the straightener. Additional back tension between the coil feed and straightening equipment also may be required due to the higher yield strength of the material in the loop as the material tries to push back against the straightener and feed system.
- Higher-strength materials, due to their greater yield strengths, have a greater tendency to retain coil set. This requires greater horsepower to straighten the material to an acceptable level of flatness. Straightening higher-strength coils requires larger-diameter rolls and wider roll spacing in order to work the stronger material more effectively. But increasing roll diameter and center distances on straighteners to accommodate higher-strength steels limits the range of materials that can effectively be straightened. A straightener capable of processing 600-mm-wide coils to 10 mm thick in mild steel may still straighten 1.5-mm-thick material successfully. But a straightener sized to run the same width and thickness of DP steel might only be capable of straightening 2.5 mm or 3.0-mm thick mild steel. This limitation is primarily due to the larger rolls and broadly spaced centers necessary to run AHSS materials. The larger rolls, journals and broader center distances safeguard the straightener from potential damage caused by the higher stresses.
- Because higher-strength materials require greater stress to blank and punch as compared to HSLA or mild steel, they generate proportionally increased snapthrough and reverse-unloading forces. High-tensile snapthrough forces introduce large downward accelerations to the upper die half. These forces work to separate the upper die from the bottom of the ram on every stroke. Insufficient die-clamping force could cause the upper-die half to separate from the bottom of the ram on each stroke, causing fatigue to the upper-die mounting fasteners.
- Because energy is expended with each stroke of the press—and this energy must be replaced—critical attention must focus on the size (horsepower) of the main drive motor and the rotational speed of the flywheel in higher-strength-steel applications. The main motor, with its electrical connection, provides the only source of energy for the press and it must generate sufficient power to meet the demands of the stamping operation. The motor must be properly sized to replace the increased energy expended during each press stroke. For these reasons, some stampers consider the benefits of servo-driven presses for these applications.
As steels becomes stronger, a corresponding increase in process knowledge is required in terms of die design, construction and maintenance, and equipment selection.
You can read more about these topics at these links:
Tooling and Die Wear
Coil Processing Straightening and Leveling
Press Requirements
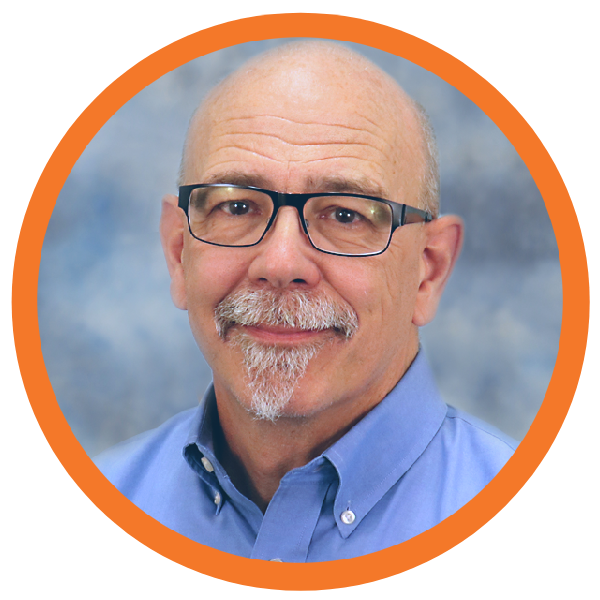
Thanks go to Peter Ulintz, of the Precision Metalforming Association (PMA) for authoring this article. Ulintz was employed in the metal stamping and tool & die industries for 38 years before joining Precision Metalforming Association (PMA) in 2015. He provides industry-related training and seminars in Stamping Press Operation and Setup; Designing and Building Metal Stamping Dies; Die Maintenance and Troubleshooting; Metal Stamping Design for Manufacturability; Deep Draw Tooling and Process Technology; Stamping Higher Strength Steels; and Problem Solving in the Press Shop. Peter is a contributor to ASM Handbook, Volume 14B, Metalworking: Sheet Forming (2006) and writes the monthly column, Tooling by Design, for PMA’s monthly publication, MetalForming Magazine.
Forming
Introduction
Approaches for forming higher strength steels evolved with the commercialization of increased strength levels of High Strength Low Alloy (HSLA) steels. Demands for greater crash performance while simultaneously reducing mass and cost have spawned the development of new groups of steels that improve on the properties of these HSLA steels. Forming of Advanced High-Strength Steel (AHSS) is not a radical change from forming conventional HSLA steels, providing some of the key differences are understood and accounted for in die design, die process, and equipment selection.
AHSS grades solve two distinct automotive needs by two different groups of steels. The first group as a class has higher strength levels with improved formability and crash-energy absorption compared to HSLA grades. DP, TRIP, FB, and TWIP steels, which have increased values of the work hardening exponent (n-value), fulfill this requirement. The second group, including CP and MS steels, extends the availability of steel in strength ranges above what is available with HSLA grades. Originally targeted for chassis, suspension, and body-in-white components, AHSS grades are now being applied to doors and other body panels. New variations in microstructure help meet specific process requirements, including increased edge stretch, bendability, strengthening after forming, or tighter property tolerances.
The progressive increases in yield and tensile strength with these new AHSS grades magnifies existing forming issues with conventional HSLA grades and creates new challenges. Concerns include higher loads on processing equipment including presses, levelers, straighteners, blanking lines, coil slitting lines and roll forming equipment. Additionally, there are material and surface treatment considerations required for tooling in the stamping plants: draw dies, trim steels, and flange steels. Compared to conventional HSLA steels, greater energy requirements result from higher AHSS yield strengths, tensile strengths and significantly higher work hardening rates. This places new requirements on press capacity, leveler, straightener and slitting capabilities, tool construction/protection, lubricant capabilities, part and process design, and maintenance. Springback management becomes more critical as yield strengths continue to increase. Conventional and press hardened (hot formed) AHSS parts have very high strength after forming, so re- forming operations should be avoided. Trimming, cutting, and piercing equipment must be constructed and maintained to overcome the extreme high strength of the final stamping. Laser cutting of press hardened parts produces a finished part that avoids pushing the limits of trim and pierce tools and dies utilized for conventional HSLA steel.
There are an ever-increasing number of AHSS multiphase microstructure grades available, each designed to resist various forming failure modes while achieving final part performance requirements. Sharing of information regarding the planned part geometry, die and stamping processing, and final part application between steel suppliers, product and die process engineering, and end users helps ensure selection of the right steel grade for the application. This becomes especially relevant since multiphase microstructures experience additional forming failure modes compared with conventional high strength products.
Tool Design Considerations
The characteristics associated with different AHSS grades influence die design and die processing decisions. Not only are these steels typically higher in strength, but they also undergo substantial work hardening during forming. These lead to increased local loads, and changes in friction, die wear, and press requirements. The multiphase microstructures increase cut edge and bending fracture sensitivity. As such, extending the life and performance of tooling in press shops requires a rethinking of tool and part design.
Part Design
Successful application of any material requires close coordination of part design and the manufacturing process. Consult product and manufacturing process engineers when designing AHSS parts to understand both the limitations and advantages of the grade and the proper forming process to be employed. Start in the concept and feasibility stage to ensure sufficient time for corrective actions and optimization.
Soft tool materials like kirksite may be used for manufacturing prototype parts and the inserts used to eliminate local wrinkles or buckles. However, wear resistant coatings are typically not applied to these tool surfaces, so the metal flow seen in these prototype parts may not match the metal flow seen under production conditions. The results from soft tool tryouts should not be used to assess manufacturability and springback of AHSS parts.
Design structural frames (such as rails, sills, cross members, and roof bows) as open-ended channels to permit forming operations rather than draw die processes. AHSS stampings requiring closed-end draw operations are limited by a reduced depth of draw, Figure 1. Less complex, open-ended stamped channels are less limited in depth. A rule of thumb is that DP 350Y600T can be formed to only half the draw depth of a mild steel.

Figure 1: Schematic of an open end part design (left) and a closed end part design (right). The open-ended design allows for greater depths when utilizing AHSS versus the closed ended design historically used with mild steel.A-5
Where possible, avoid closed-end developments to make more complex geometries with AHSS grades. Wrapping ends of “hat” sections increases forming loads, increases the chances of circumferential compression wrinkling on the binder, specifically in the corners, and increases wrinkling on the draw wall if the blank edge runs through the draw bead. Draw die developments that include a closed (or wrapped) end development usually also require a larger blank size. During draw die development, it is best to identify parts that have a “hat” section geometry in certain locations and develop the draw die accordingly to maximize the positive formability attributes of AHSS while minimizing the limitations of AHSS.
For example, the left image in Figure 2 shows a draw die development on a DP600 cowl side with a closed (wrapped) end, with the right image showing a similar part developed with an open end. Although both final part geometries are similar, the closed-end development led to significant global formability failures due to the excessive stretch. In contrast, the open-ended development had virtually no global formability related failures. Other design and die development differences in the part on the right include the use of stake beads to control springback and embossments to eliminate wavy metal. In addition, an open-ended development has the potential to reduce the blank size for material utilization savings.

Figure 2: Draw die development for a cowl side formed from DP600. Left image: closed-end development with global formability failures, waviness, and springback. Right image: open-ended development with no splits, waves, or dimensional concerns.U-6
The automotive industry has adopted a strategy for “lighter dies and fewer dies”, to reduce cost. One key element is “part consolidation”, such as one-piece body side outers and inners. High strength steels challenge the part consolidation mantra. When encountering extreme formability challenges, parts previously made with one set of dies when stamped from lower strength steels may benefit from transitioning to a laser welded blank with a lower strength grade in the challenging region and higher strength steels in the remainder of the part. Alternatively, splitting the consolidated part into two or more separate parts subsequently welded together may improve stamping success at the expense of another operation. In the past, one-piece rocker panels were stamped from conventional mild or HSLA steel. However, this component requires higher strength and reduced thickness to meet weight and crash requirements, so now DP980 is often considered as the grade of choice for this application. Figure 3 shows a rocker panel where insufficient formability of DP980 prevented a one-piece stamping. The OEM solved this by dividing the part into two stampings, putting a more formable grade where needed on the wrapped (or closed) end.

Figure 3: When a one-piece rocker panel could not be successfully formed from DP980, the OEM stamped a DP980 rocker panel section with an open-ended design and spot welded it to a mild steel end cap.U-6
Trim and Pierce Tool Design
- Trim and pierce tools need to withstand higher loads since AHSS grades have higher tensile strengths than conventional high-strength steels.
- Edge cracking is minimized with proper support of the trim stock during trimming.
- Modify timing of the trim/pierce operation to minimize snap-through reverse loading.
- Scrap shedding may be an issue, since AHSS springback can cause scrap to stick in the tool.
Flange Design
- Design more formable flanges to reduce need for extra re-strike operations.
- Areas to be flanged should have a “break-line” or initial bend radius drawn in the first die to reduce springback.
- Adapt die radii for material strength and blank thickness.
Draw Bead Design
- Metal flow across draw beads generates strain and minimizes the elastic recovery which causes springback.
- Metal flow across draw beads generates large amounts of work hardening, leading to increased press loads.
- Optimizing blank size and shape reduces the reliance on draw beads, which can excessively work harden the material before entering the die opening.
Guidelines to Avoid Edge Cracking During Stretch Flanging
- Flange length transition should be gradual – abrupt changes in flange length cause local stress raisers leading to edge cracks.
- Use good cutting practices to achieve a high-quality edge.
- Avoid the use of sharp notch features in curved flanges.
- Avoid putting bypass notches in stretch or compression edges of blanks or progressive die carrier strips. These bypass notches can act as stress risers and lead to edge fractures in the draw or flange operation. In addition, bypass notches in blanks and progressive dies are difficult to maintain, which can increase the potential for edge fracture.
- Metal gainers in the draw die or in the die prior to the stretch flange operation compensates for change in length of line that occurs during flanging, helping to avoid edge cracking. In the example shown in Figure 4, edge fractures moved from the draw panel to flanged panel after grinding on the draw die to eliminate edge fractures in the draw operation. The draw panel underneath the flanged part in Figure 4 did not have edge fractures. The reduction in the length of line in the draw operation moved the problem to the flanged part where the stamping transitioned from bending and straightening in the flange operation to a stretch flange operation. A better practice is to add metal gainers to the draw panel to provide the feedstock which expands during stretch flanging.

Figure 4: Flanged panel fractures, with the draw panel underneath. Adding metal gainers to the draw panel would help minimize these fractures.U-6
- The higher strength of AHSS makes it more difficult to pull out loose metal or achieve a minimum stretch in flat sections of stampings. Addendum, metal gainers (Figures 5 and 6), and other tool features balance lengths of line and locally increase stretch.

Figure 5: Metal gainers help avoid insufficient stretched areas and eliminate buckles.T-3

Figure 6: Metal gainers and depressions balance stresses and minimizes wrinkled metal.A-41
Tooling
topofpage
Tool and die wear occurs due to the friction produced from the contact between the sheet metal and the tooling surface. Damage to the die surface can cause a gradual loss of tooling material, and scoring or burnishing damage to the sheet metal surface may be stress risers leading to premature failure in formed parts.
Impacting tool wear are the die material, strength of sheet metal, contact pressure, surface finish of the sheet and tooling, sliding velocity, temperature, coating of the die, and lubrication used. Advanced steel grades, where work hardening during stamping further increases the strength of an already high strength product, may result in additional die wear. Die wear beyond a critical point calls for replacement of the current die, impacting turnaround times and to production losses.
New die materials and better die coatings exist which minimize the impact of excessive tooling wear when forming AHSS. These new die materials include wrought and cast tool steels as well as powder metallurgy tool steels, which retain hardness without compromising the toughness of the material. Furthermore, hard material coatings and nitriding can improve the tribological properties of die surfaces.
Most tool materials for sheet metal forming are cast iron, cast steel, or tool steels.N-12 Cast iron grades used for stamping applications are gray cast irons (like G2500, G25HP and G3500) and pearlitic ductile irons (D4512, D6510, and D7003, among others). Cast steel grades include S0030, S0050A, S7140, and S2333. Tool steels include TD2 (a high wear / low shock resistant tool steel), TS7 (a high shock / low wear resistant tool steel) and TA2 (a balanced medium wear / medium shock resistant tool steel). These designations come from Reference NAAMS, with other designations in Citations A-37, A-38, I-11, J-7, J-8, J-9 Many of these designations overlap and represent the same or highly similar product. For example, ASTM A681 D2, JIS G4404 SKD11, and ISO 4957 X153CrMoV12 cover the same alloy tool steel.
In general, existing tool and die shop procedures to select the appropriate die material are applicable to select dies made to stamp Advanced High Strength Steels. However, the considerably higher strength level of these grades exerts proportionally increased load on the die material. AHSS grades might reach hardness values 4 to 5 times higher than mild steel grades. This is partially due to the microstructure of the sheet metal itself since some grades achieve higher strength from the microstructural phase martensite. Some martensitic grades (MS) have a tensile strength higher than 2000 MPa. This strength level corresponds to Rockwell C values higher than 57, meaning that the sheet metal hardness is approaching the tooling hardness.
The higher forces required to form AHSS require increased attention to tool specifications. The three primary areas are:
- Stiffness and toughness of the tool substrate for failure protection.
- Harder tool surface finishes for wear protection.
- Surface roughness of the tool.
The accepted amount of wear/galling between maintenance periods is a key factor in determining the performance requirements of draw dies, punches, and other tooling components. Some of the key elements that affect the die material specification include:
- The chosen sheet metal to be processed, as characterized by strength, thickness, surface coating, and surface profile (roughness and peak count).
- Die construction, machinability, radii sharpness, surface finish, and die hardness, specifically on draw beads and radii.
- Lubrication.
- Targeted cost per part.
Counteracting the increased applied load required to form AHSS grades is a potential reduction in sheet thickness. This thickness reduction leading to lighter weight parts is one of the key drivers promoting expanded use of Advanced High Strength Steels. Unfortunately, the reduced thickness of the steel increases the tendency to wrinkle. Suppressing these wrinkles requires higher blankholder forces. Any formation of wrinkles will increase the local load and accelerate the wear effects. Figure 1 shows a draw die with severe die wear due to excessive wrinkling on a DP980 part. It is not uncommon to replace these high wear areas with a more durable tool steel insert to minimize this type of excessive wear condition.

Figure 1: Draw die with significant wear due to excessive wrinkling on a DP980 part. S-45
Surface Hardening Treatments and Coatings
Surface treatments and coatings help increase tool life and reduce friction. Flame or induction hardening heat treatments, nitriding, and chrome plating are common surface treatment techniques used. However, each of these can fail under the high contact pressure that is present when stamping advanced high strength steels. Coating the inserts adds additional wear and friction benefits.
Many surface hardening options exist which improve the wear resistance.A-7 Carbon content limits the achievable surface hardness with either flame hardening or induction hardening. Tools hardened with either approach must be quenched after heating, which increases the risk of distortion. Laser beam hardening relies on the high thermal conductivity of underlying base tool steel to self-quench, which reduces the magnitude of distortion. Further minimizing distortion: the energy input in laser beam hardening is approximately 10% of flame hardening.
Carbon and nitrogen increase the strength and hardness of sheet steels. Similarly, carburizing and nitriding tool steels create a hard, wear-resistant surface layer. Carburizing is done at a higher temperature, which carries the risk of distortion. Nitriding takes primarily one of two forms: gas nitriding and plasma (ion) nitriding. Ion nitriding is faster than gas nitriding, accomplished at a lower processing temperature, and minimizes the thickness of the brittle “white layer.” A-39
Chrome plating of tools and dies has been an option to increase wear resistance, but may exhibit microcracking. Environmental concerns further limit its use. In addition, studies show that is it not the best option for tools used to form advanced high strength steels.Y-6
A high hardness, low friction coating results in a wear resistant surface that lowers the risk for galling. Coatings include titanium nitride (TiN), titanium carbide (TiC), titanium carbonitride (TiCN), titanium aluminum nitride (TiAlN) and chromium nitride (CrN). Common application methods are physical vapor deposition (PVD), chemical vapor deposition (CVD), and thermal diffusion (TD).
The strength of metallurgical bonds produced in the CVD and TD processes are greater than physical bond associated with the PVD approach. However, application of CVD and TD coatings occurs at around 1000 degrees C, which is likely in the austenite region of the tool steel. This high temperature can soften the die, which then necessitates a subsequent rehardening process, and may also cause dimensional distortion. For these reasons, several global automakers specify only PVD coatings.
The benefits of PVD coatings in reducing galling are apparent in Figure 2, which compares a cut edge after blanking of 200,000 parts of CR 500Y/800T-DP. Use of cutting steels with a PVD-applied TiAlN coating results in a cleaner, more uniform edge.
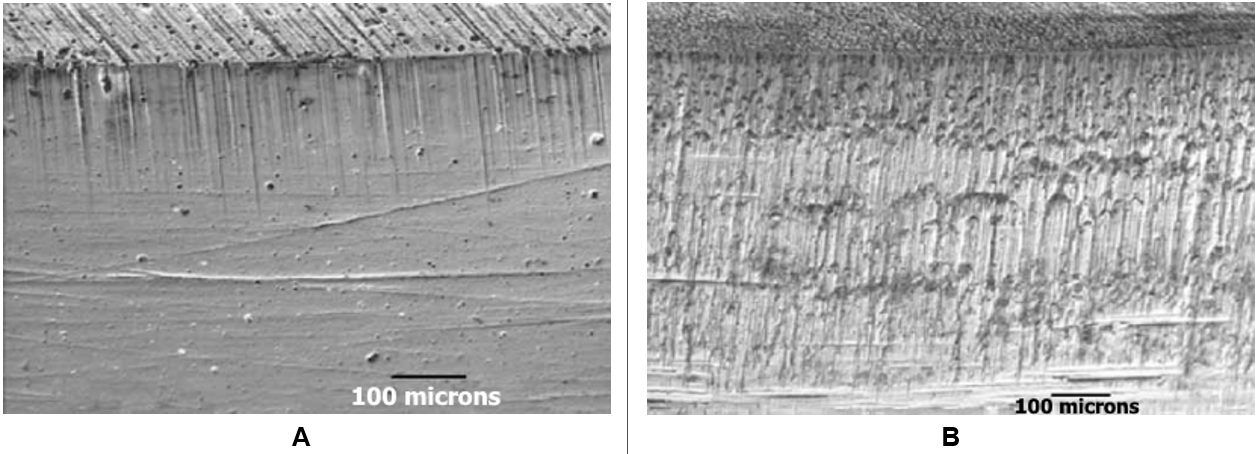
Figure 2: PVD-applied TiAlN-coated cutting steel (image A) reduces galling compared with an uncoated cutting steel (image B). Edges are shown after 200,000 parts produced from CR 500Y/800T-DP. T-20
Since coatings may crack, it is important that the substrate has sufficient hardness/strength to avoid even the slightest plastic deformation of the tool surface. Therefore, the recommended practice is to perform an initial surface hardening treatment, typically flame or induction hardening followed by ion nitriding, to develop substrate hardness and strength before applying the coating. Surface roughness must be as low as possible before coating, with average surface roughness (Ra) values below 0.2 μm recommended. This roughness level approximates a 600-grit sandpaper finish.
Considering the high cost of coated tool steels, a recommended approach is to construct large forming tools from relatively inexpensive and soft materials, such as cast iron or low-grade tool steel. Locations subject to severe wear are candidates for inserts of high-grade tool steels with an appropriate coating engineered for the application.
Ceramic tool inserts have extreme hardness for wear resistance, high heat resistance, and optimum tribological behavior, but have poor machinability and severe brittleness. Potentially offsetting the higher cost are reduced maintenance and increased productivity. While not commonly used, the ceramic tool inserts offer a possible solution to high interface loading and wear.
Select tool steel inserts for forming dies according to the sheet metal and the forming severity. These inserts should have a surface coating when processing DP 350/600 and higher grades. Initial tryout should be completed before coating, so that die adjustments and springback compensation efforts do not lead to removal of the newly applied coating. Allow for tooling recuts during this tryout loop to ensure the resulting tool has sufficient mass and stiffness. Different friction and metal flow conditions should be expected between the initially uncoated and the ultimately coated tool steels.
The optimal surface treatment may increase upfront cost, but will reduce the rework and die maintenance cost over the life of the die. Shown in the top two lines of Figure 3 are the benefits of plasma ion nitriding a flame hardened graphite-bearing cast iron tool (GGG70L) when forming 1 mm thick electrogalvanized dual phase steel. The bottom two lines show the effect on AISI D2 (DIN 1.2379 or JIS SKD11), highlighting only minimal tooling wear over the 5000 parts evaluated.

Figure 3: Plasma ion nitriding leads to reduced tool wear. GGG70L is a flame hardened spheroidal graphite-bearing cast iron and DIN 1.2379 is AISI D2 or JIS SKD11 cold work tool steel.T-11
Figure 4 compares the surface appearance of the same tool steel with different coatings. On the left is a chrome-plated tool which exhibited adhesive and abrasive wear, and ran for only 50,000 parts. Shown on the right is an ion nitrided tool steel which was chromium nitride coated using PVD, and produced more than 1.2 million parts.

Figure 4: Tool steel surface. Left image: Chrome plated, failed after 50,000 parts; Right image: Ion nitride tool steel, chromium nitride PVD coating, produced more than 1.2 million parts. J-10
Heat produced from stamping AHSS grades interacts with the tool material and coating, which may impact friction and metal flow. 1mm thick hot dip galvannealed CR340Y/590T-DP-GA was evaluated in a laboratory set-up.S-46 Initially at room temperature, die surface temperature increased to 65 °C (150 °F) after 10 cycles of passing this DP590 grade across a tool radius and significant zinc powdering occurred. Less powdering occurred with the use of a die coolant. Cooling the dies also helped to reduce the surface scoring and associated friction [Figure 5].
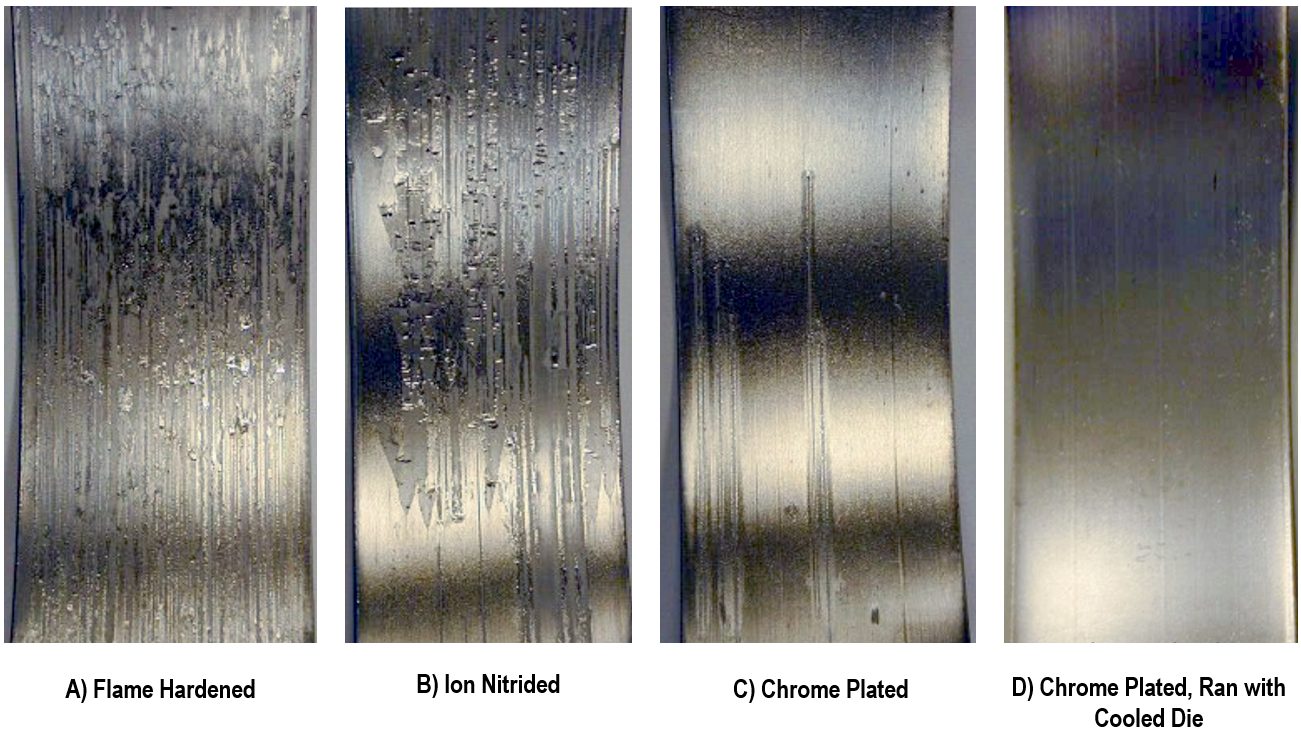
Figure 5: Coatings and Die Coolant on D2 Tool Steel Reduce Scoring and Friction with galvannealed dual phase steel. A) Flame Hardened; B) Ion Nitrided; C) Chrome Plated; and D) Chrome Plated with die coolant.S-46
Selection of tool steels for cutting, trimming, and punching tools have similar considerations as forming tools. The base tool steel must have excellent chipping and cracking resistance. Coatings will reduce tool wear. Hardening of the substrate prior to coating will minimize failure due to plastic deformation of the substrate. Coatings reduce the severity of the shock wave produced when cutting advanced high strength steels. See the Cutting / Blanking / Shearing / Trimming page for more information.
It is not advisable to use only one tooling solution for all Advanced High-Strength Steels. One study showed that die material and coating methods used in large volume production of steel grades up to and including those with 980 MPa minimum tensile strength were not suitable for forming a grade with 1180 MPa minimum tensile strength.W-18 Furthermore, this study recommends avoiding die materials such as ductile iron and low alloy cast steel when stamping 1180 grade steels.
In addition to selecting the correct die material, it must be processed appropriately. Figure 6 shows the effects of proper heat treatment when stamping a dual phase steel with 980MPa minimum tensile strength.S-45 This same study showed that a PVD coated tool performed best when forming a DP steel without a galvanized coating, yet the PVD coating led to significant zinc buildup when forming a galvanized DP steel. An ion nitride tool coating worked best for galvanized steels.
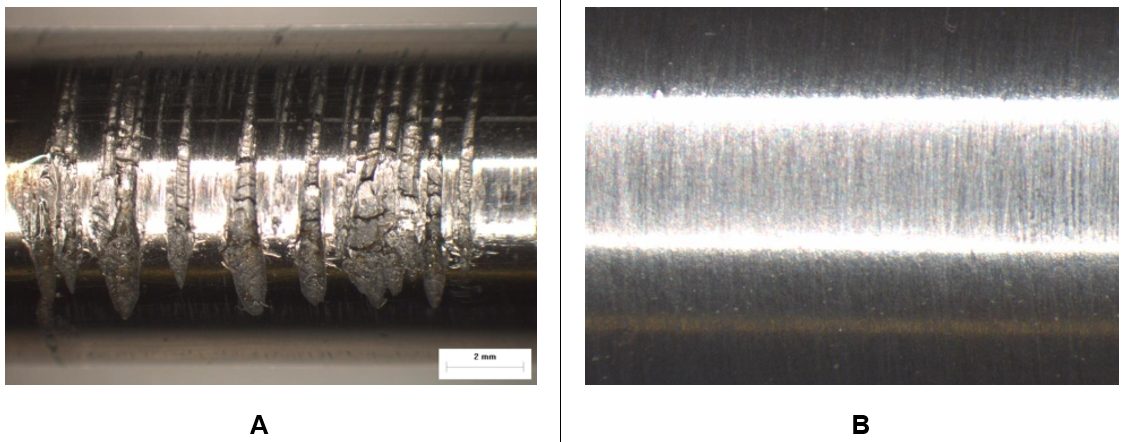
Figure 6: Effect of Heat Treatment on Tools to Stamp Dual Phase Steel. Image A) No heat treatment leads to wear; Image B) Proper heat treatment results in a surface without damage.S-45
There are five main types of cold work failure modes involving tool steels – wear, plastic deformation, chipping, cracking, and galling. There is also interaction between these failure modes. Figure 7 shows examples of these failure modes.T-20, U-7
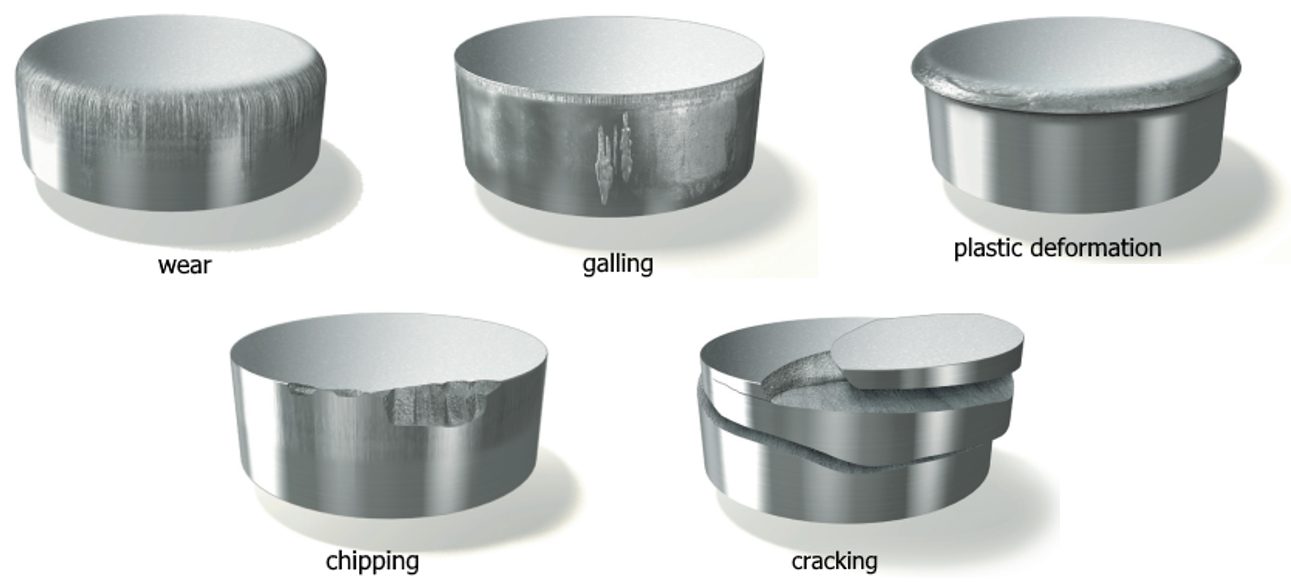
Figure 7: Stamping Tool Failure Modes. T-20, U-7
Wear is damage to the tooling surface resulting in material loss and is related to the tooling material hardness, and the type, volume, and distribution of hard particles like oxides or carbides. Wear can also be related to material type and process conditions and involves sliding contact between the tooling and the material. There are two types of wear: abrasive and adhesive.
Abrasive wear occurs when hard particles forced into a surface during the sliding contact leads to removal of metal from the tool steel. Tool steel properties promoting abrasive wear resistance include high hardness of the tool steel and of the carbides, as well as a high volume of large carbides. However, the high hardness targeted for wear resistance makes the material sensitive to notches. Large carbides act as crack initiators, increasing the risk of fatigue cracking.
Adhesive wear occurs with material transfer from one metal surface to another. The friction and heat generated as the sheet metal slides across the tool surface results in micro-welding between the asperities (peaks) on each surface. Failure of these micro-welds occurs with continued relative motion between the two surfaces, with small fragments torn from the weaker side surface and adhering to the other surface. The material ripped out of the tool steel will occasionally stick to the sheet metal surface. With continued metal motion, these pieces may score and damage the tool steel surface resulting in a combination of adhesive and abrasive wear known as mixed wear.
Galling is a physical/chemical adhesion of the sheet metal to the tool surface. The severity of the galling depends on the surface finish and chemical composition of the material and the tool steel and involves the friction and sliding contact between the tooling and the material. Galling, abrasive wear, and adhesive wear are related, and can be minimized through the use of proper surface treatments or coatings on top of a tool steel with high hardness (Figure 8).

Figure 8: Adhesive wear of the tooling leads to abrasive wear scratches on the DP600 sheet surface. Continued abrasive wear leads to galling of the sheet surface. Higher magnification images are shown on the bottom. G-18
Plastic deformation occurs when the stress from contact with the sheet metal exceeds the compressive yield strength of the tool material. A high hardness tool steel helps to avoid this damage.
Chipping occurs when the operating stress levels exceed the fatigue strength of the tool steel, typically found at sharp edges. Microcracks initiate in the high contact area of the tool surface, propagate, and ultimately result in pieces chipping out along edges or at corners. Chipping may initiate in areas affected by adhesive wear. Here, microcracks can nucleate, deepen, and spread, leading to a fatigue failure. A tool steel with high ductility has good chipping resistance, since microcrack initiation and propagation are more difficult.
Cracking occurs when the operating stress levels exceed the fracture toughness of the tool material. Crack formation occurs in the presence of stress concentrators, like grinding and machining marks or design features such as sharp corners or radii. Once the crack forms, unstable crack propagation leads to failure. Microstructural toughness promotes good cracking resistance, as does low hardness. However, low hardness has a detrimental effect on the resistance to the other failure mechanisms and is not normally a good solution.
Higher strength steels lead to greater demands on the wear resistance and mechanical strength of the tool material. Forming operations require high wear and galling resistance and compressive strength. Cutting operation require a combination of high wear resistance, high galling resistance, high compressive strength, high chipping, and total cracking resistance.
Tool materials must balance compressive strength and toughness with resistance to wear, thermal, and mechanical stresses.
Conventional highly alloyed tool steels are produced from large ingots. The slow solidification leads to microstructural segregation, forming large carbide networks which turn into carbide stringers after processing. These networks are beneficial for wear resistance, but reduces fatigue strength and toughness.
Alternate approaches minimizing segregation reduces these concerns. Two such production methods are electroslag remelting and powder metallurgy.T-20
Electroslag remelting (also known as electroslag refining, ESR) is a progressive melting process used to produce porosity-free ingots of uniform chemistry. Under a protective atmosphere, only a small portion of the ingot is liquid at any one time, and solidification occurs in a controlled manner. This processing approach results in tool steels with increased cleanliness, smaller carbides, and improved ductility and fatigue properties. It is relatively expensive, leading to its use in some specialized tool steel applications.
Rather than slowly solidifying in a large ingot, powder metallurgy (PM) production involves first atomizing a stream of molten metal using a high pressure inert gas, resulting in droplets that rapidly solidify into powder. Segregation is typically a fraction of the powder diameter, which is on the order of 100 μm. Hot isostatic pressing (HIPing) consolidates the collected powders, which is subsequently rolled or forged in a similar manner used for ingots.
Without the concern of macro-segregation or large carbides, the PM approach allows for manufacturing of more highly alloyed tool steels than is possible with conventional ingot metallurgy. Here, the carbides are smaller and more evenly distributed even compared with the ESR approach, leading to a balance of wear resistance and fatigue life. PM tool steels have enhanced resistance to abrasive wear, adhesive wear, chipping, and cracking. Coatings improve galling resistance.
Metal stampers and die shops experienced with mild and HSLA steels often have problems making parts from AHSS grades. The higher initial yield strengths and increased work hardening of these steels can require as much as four times the working loads of mild steel. Some AHSS grades also have hardness levels approaching the dies used to form them.
The higher stresses required to penetrate higher-strength materials require increased punch-to-die clearances compared to mild steels and HSLA grades. Why? This clearance acts as leverage to bend and break the slug out of the sheet metal. Stronger materials need longer levers to bend the slug. The required clearance is a function of the steel grade and tensile strength, and sheet thickness.
Increasing cutting clearance can result in punch cracking and head breakage due to higher snapthrough loads and reverse-unloading forces within the die. Adding shear angles to the punch face helps reduce punch forces and reverse unloading.
Tight cutting clearances increase the tendency for die galling and chipping. The severity of galling depends on the surface finish and microstructure of both the tool steel and work material. Chipping can occur when process stresses are high enough to cause low-cycle fatigue of the tooling material, indicating that the material lacks toughness.
Tempering of tools and dies represents a critical heat-treatment step and serves more than one purpose, but of primary concern is the need to relieve residual stresses and impart toughness. Dies placed in service without proper tempering likely will experience early failure.
Dies made from the higher-alloy tool-steel grades (D, M or T grades) require more than one tempering step. These grades contain large amounts of retained austenite and untempered martensite after the first tempering step and require at least one more temper to relieve internal stresses, and sometimes a third temper for even greater toughness.
Unfortunately, heat treatment remains a “black-box” process for most die shops and manufacturing companies, who send soft die details to the local heat treat facility, with hardened details returned. A cursory Rockwell hardness test may be conducted at the die shop when the parts return. If they meet hardness requirements, the parts usually are accepted, regardless of how they may have been processed—a problem, as hardness alone does not adequately measure impact toughness.
Consider this scenario: An automotive structural part has been in production for years as a conventional high strength steel with a minimum yield strength of 280 MPa, CR280Y350T-LA. In order to meet increasing global safety regulations, the automaker converts the part to a dual phase steel, CR340Y590T-DP. Even though these grades have relatively close minimum yield strength levels as produced at the steel mill, dual phase steels have excellent work hardening characteristics and are bake hardenable. These are among the reasons for their favorable response in crash events in comparison to HSLA grades.
The stamping location attempted to do a direct swap, substituting the DP steel for the HSLA grade with no changes to the part or process. Immediately after the grade change, scrap rates increased significantly. The failures were all determined to be local formability edge fractures; investigation revealed that the edge of the configured blank remained as the final product edge which split during forming and subsequent flanging. Examination of the edge revealed a burr as well as a non-uniform cut edge appearance. The tool showed signs of chipping.
Issues found, along with corrective actions:
- The tool steel used for HSLA (uncoated D2) was not appropriate for stamping DP590. Upgrade to a more durable tool steel with good chipping resistance. Add a surface treatment or coating if needed for additional wear resistance.
- The stamper used the traditional 10% tooling clearance when blanking HSLA. The recommended clearance for DP590 at the thickness studied is 15%.
- The flanging operation further expanded the edge beyond what occurred in the draw die. DP steels work harden to a much greater degree than HSLA steels. As such, stretch flanging a cut edge significantly increases the potential for edge fracture in this stronger product. Adding a metal gainer to the draw die ensures the flanging operation performs only bending and straightening, and does not further stretch a cut edge.
Making these changes to accommodate the new grade eliminated scrap from this process.
Upgraded Sheet Metal Forming (M-20)
Multi-phase steels are complex to cut and form, requiring specific tooling materials. The tooling alloys which have been used for decades, such as D2, A2 or S7, are reaching their load limits and often result in unacceptable tool life. The mechanical properties of the sheet steels achieve tensile strengths of up to 1800 MPa with elongations of up to 40%. Additionally, the tooling alloys are stressed by the work hardening of the material during processing.
The challenge to process AHSS quickly and economically makes it necessary for suppliers to manufacture tooling with an optimal tool steel selection. The following case study illustrates the tooling challenges caused by AHSS and the importance of proper tool steel selection.
A manufacturer of control arms changed production material from a conventional steel to an Advanced High-Strength Steel (AHSS), HR440Y580T-FB, a Ferrite-Bainite grade with a minimum yield strength of 440 MPa and a minimum tensile strength of 580 MPa. However, the tool steels were not also changed to address the increased demands of AHSS, resulting in unacceptable tool life and down time.
According to the certified metal properties, the 4 mm thick FB 600 material introduced into production had a 525 MPa yield strength, 605 MPa tensile strength, and a 20% total elongation. These mechanical properties did not appear to be a significant challenge for the tool steels specified in the existing die standards. But the problems encountered in production revealed serious tool life problems.
To form the FB 600 the manufacturer used D2 steel. D2 was successful for decades in forming applications. This cold work tool steel is used in a wide variety of applications due to its simple heat treatment and its easily adjustable hardness values. In this case, D2 was used at a hardness of RC 58/60.
While tools manufactured from D2 can withstand up to 50,000 load cycles when forming conventional steels, these particular D2 tools failed after only 5,000 – 7,000 cycles during the forming of FB 600. The first problems were detected on a curl station where mechanical overload caused the D2 tools to break catastrophically, as seen in Figure 9 below. Since the breakage was sudden and unforeseeable, each failure of the tools resulted in long changeover times and thus machine downtime.

Figure 9: Breakage seen in control arm curl tool made from D2, leading to premature failure. Conversion to a PM tool steel having higher impact resistance led to 10x increase in tool life.M-20
Since the cause of failure was a mechanical breakage of the tools, a tougher alternative was consequently sought. These alternatives, which included A2 and DC53® (a registered trademark of International Mold Steel) were tested at RC 58-60 and unfortunately showed similar tool life and failures.
Metallurgical analysis indicated that the failure resulted from insufficient impact strength of the tool steel. This was caused by the increased cross-cut that the work-hardened AHSS exerted on the curl. As an alternative material, a cold work steel with a hardness of 58-60, a tensile strength of about 2200 – 2400 MPa and high toughness was sought. These properties could not be achieved with conventional tool steels. The toolmaker used a special particle metallurgy (PM) tool steel to obtain an optimum combination of impact strength, hardness and wear resistance.
Particle metallurgy (PM) tool steels, due to their unique manufacturing process, represent improvements in alloy composition beyond the capabilities of conventional tool steels. Materials with a high alloy content of carbide formers such as chromium, vanadium, molybdenum and tungsten are readily available. The PM melting process ensures that the carbides are especially fine in particle size and evenly distributed (reference Table 1). This process results in a far tougher tool steel compared to conventional melting practices.

Table 1: Elemental Composition of Chosen Tool Steel
The manufacturer selected Z-Tuff PM® to be used at a hardness of RC 58-60. Employing the identical hardness as the conventional cold work steel D2, a significant increase in impact strength (nearly 10X increase as measured by un-notched Charpy impact values) was realized due to the homogeneous microstructure and the more evenly distributed precipitates. This positive effect of the PM material led to a significant increase in tool life. By switching to the PM tool steel, the service life is again at the usual 40,000 – 50,000 load cycles. By using a steel with an optimal combination of properties, the manufacturer eliminated the tool breakage without introducing new problems such as deformation, galling, or premature wear.
AHSS creates tooling demands that challenge the mechanical properties of conventional tool steels. Existing die standards may not be sufficient to achieve consistent and reliable performance for forming, trimming and piercing AHSS. Proper tool steel grade selection is critical to ensuring consistent and reliable tooling performance in AHSS applications. Powder metallurgical tool steels offer a solution for the challenges of AHSS.
- Areas seeing higher working loads require improved tool materials and coatings for both failure protection and wear protection.
- The higher initial yield strengths of AHSS, plus the increased work hardening of DP and TRIP steels can increase the working loads by 400% compared to Mild steels.
- Strength levels of some AHSS grades are associated with the same hardness as the tools intended to form them.
- Advanced powder metallurgy (PM) tool materials are appropriate for some AHSS applications.
- The dominant mode of failure of tool steels should be identified and communicated to the tool steel supplier to select the tool steel with the best properties to combat the tool failure mode for a given AHSS grade.
- PVD coated tool steels are appropriate for stamping AHSS grades without a galvanized coating but may result in zinc buildup when forming galvanized steels. Here, an ion nitride coating may be appropriate.
- Complete initial tryout before application of tool coating. Allow for tooling recuts during tryout loop to ensure the resulting tool has sufficient mass and stiffness.
- Proper selection of tool material, surface treatment, and coating leads to a reduction in maintenance, repair, and scrap/rework costs. Further offsetting the upfront tooling costs are improved process uptime, improved quality, and greater consistency.
Back to the Top
Tooling
Lubrication is a key input into the sheet metal forming process. The chosen lube, application method, distribution, and cleaning method influences forming and subsequent operations.
Lubricant Functions and Requirements
Lubricants serve many roles in sheet forming. The functions below become more challenging as the strength of the sheet metal increases, resulting in higher forces, contact pressures, and temperatures. Therefore, special attention to lubrication and the effect of heat are required when considering lubricants for forming Advanced High-Strength Steels (AHSS).
- Control metal flow from the binder.
- Redistribute strains over the punch.
- Maximize/minimize the growth of strain gradients (deformation localization).
- Reduce surface damage from adhesive die wear (galling and scoring).
- Remove heat from the deformation zone.
- Change the influence of surface coatings.
- Prevent corrosion on stamped parts prior to finishing.
- Remain removable after parts storage.
- Must be safe in use and sustainably produced.
AHSS Energy, Heat and Lubrication
Higher strength steels (both conventional and AHSS) have less capacity for stretch (less work hardening or n-value than mild steels) over the punch to generate the required length of line. As the steel strength increases, more metal must flow from the binder into the die to compensate for the loss of length of line for a required stamping depth. Tensile stresses are applied to the metal under the binder in the radial direction (perpendicular to the die radius) to pull the metal towards the die radius. Compressive stresses can form in the circumferential direction (parallel to the die radius) as the blank reduces its circumferential length. While this compression usually happens in box corners, it also can happen in sidewall features that shorten the length of line while moving into the stamping. Metal flowing uncontrolled into a sidewall also can generate compressive circumferential stresses. These compressive stresses tend to buckle the binder metal rather than uniformly increase the local thickness. This buckling is following the law of least energy-forming mode in sheet metal forming. Less energy is required to form a local hinge (a buckle) using only few elements of the sheet metal compared to uniformly in-plane compressing the metal to generate an increase in thickness for a large number of elements.
Weight reduction programs use higher strength steels to reduce the sheet metal thickness. The thinner sheet metal is more prone to buckling than thicker steels. Therefore, part designs utilizing AHSS with thinner sheets can require significantly higher blankholder forces to flatten buckles that form. Since restraining force is a function of the coefficient of friction (C.O.F.) multiplied by the blankholder force, the restraining force increases and metal flow decreases. Deforming AHSS requires more energy. This combination results in higher contact pressure between the metal and the die, and higher interface temperatures. Counter measures include an improvement in lubrication with a lower coefficient of friction, and the ability to maintain viscosity at elevated temperatures; other process changes may be required to compensate for the pressures and temperature effects.
Higher forming energy causes both the part and the die to increase in temperature. For example, a study by IrmcoJ-16 measured the temperatures on stampings produced from 4mm thick 350 MPa and 560 MPa steels, with a production rate of either 10 or 13 strokes per minute. Increasing strength levels result in higher part temperatures, which is made more significant at higher stroke rates, Figure 1. The die temperatures are also significantly higher, and conventional water-based and oil-based lubricants may suffer viscosity reduction, with a corresponding increase in the coefficient of friction.

Figure 1: Part and tooling temperatures increase when stamping higher strength steels.J-16
Several lubrication studies have been performed over the years, including Citation P-11 and many others from The Center for Precision Forming (CPF) at Ohio State University.K-19, H-24, F-13 Figure 2 shows the temperature profile after a single stroke of deep drawing a 1.2-mm-thick DP980 part (470 by 300 mm) in a 300-ton AIDA servo press with CNC hydraulic cushion.F-28

Figure 2: Temperature profile after deep drawing a 1.2mm DP980 part in a servo press with a hydraulic cushion.F-28
The highest temperatures exceeding 200 °C are found on the die opening radius – reinforcing that part and die temperatures increase with increasing material strength. Without high-temperature tolerant additives, the lubricant effectiveness deteriorates, resulting in galling and subsequent scoring of the sheet metal. All these events increase blankholder-restraining force and inhibit metal flowing into the die. As production speed increases (the number of strokes per minute), the amount of heat generated increases, with a corresponding increase in sheet metal and die temperature.
One approach to addressing the heat problem when forming higher strength steels is application of lubricants that are less prone to viscosity changes with temperature, and lubricant film breakdown. Water-based lubricants disperse more heat than oil-based lubricants. Successful forming of some parts may require tunnels drilled inside the tooling for circulating cooling liquids. These tunnels target hot spots (thermal gradients) that tend to localize deformation leading to failures.
Lubricant Selection
The metalforming lubrication system is complex, affected by numerous factors.H-25 The information provided here is a general overview, but it is recommended that personnel selecting lubricants consult their lubricant supplier(s) regarding the specific requirements of each application.
TribologyA-50 is the science of friction, lubrication, wear, and erosion. Triboelements are the interacting surfaces in a tribosystem; the tool and workpiece in sheet metal forming. A tribosystem is any system containing triboelements, including all mechanical, chemical, and environmental factors relevant to the tribological behavior. These include the materials and surface properties, contact geometry, loading, motion, and environment. All these factors may influence the coefficient of friction. Coefficient of friction (µ) is the ratio of the force required to move one surface over another contacting surface (F) to the applied normal force (P).S-62
µ = F/P
In forming of High-Strength Steels (HSS), the combination of high interface pressures, sliding contact, and expanding surfaces resulting in lubricant starvation makes boundary lubrication particularly important in preventing lubricant film failure and adhesion or galling. Boundary lubrication is when the coefficient of friction is a function of the properties of the surfaces in contact, and the lubricant, other than viscosity.O-5 Polar boundary lubricants adhere to the surfaces, forming thin films, which prevent or delay adhesion and galling. Extreme pressure (EP) is a type of boundary lubrication where the EP additive, typically chlorine, sulfur, or phosphorus bearing, reacts with the metal surface, forming metallic salts, along with other reaction products, which provides a lubricating barrier (tribofilm) between the surfaces. These are generally thought to be effective up to the melting point of the metallic salt. For phosphorus, this is about 205 °C, chlorine 700 °C, and sulfur 960 °C. A typical polar organic boundary lubricant like fatty acid soaps are generally not effective much above 100 °C.M-23 EP additives are often combined in lubricant formulas along with other boundary lubricants to optimize performance across a range of conditions and take advantage of synergies. Chloride salts on the metal surface makes rust protection more challenging with chlorinated EP lubricants. This factor and continuous regulatory pressure have resulted in the decreasing use of chlorine-bearing additives.
Initial activation of EP lubricants was previously believed to be almost completely thermally driven, with graphs like Figure 3L-32 indicating activation and breakdown temperatures. It has since been shown that initial activation of boundary additives depends on both the applied shear stress and temperature, and is the subject of ongoing research.S-69, A-53,Z-8 For operations above these temperatures, non-reactive inorganic solids may be used, including graphite, molybdenum disulfide, calcium carbonate, and nanoparticles.

Figure 3: Activation and breakdown temperature of various additives used in metalforming lubricants.L-32
Liquid metalforming lubricants may be classified by the amount of mineral oil contained within them.B-35, A-54
- Straight Oils: Mineral oil based and used as received.
- Soluble Oils or Emulsifiable Oils: 50-80% oil, emulsified in water prior to use.
- Pre-formed Emulsions (Semisynthetic): These may contain 3% to 30% mineral oil. They are ready to use emulsions blended by the lubricant manufacturer. These are usually used as received, but may be diluted further in water for use.
- Water-Based Synthetics: These contain no mineral oil, are water based as received, and may be used as received or diluted further for use.
Lubricants used to form HSS may be further classified according to their application and how they are used.B-34
- Mill oil: Corrosion preventative oil applied at a sheet metal mill or processor providing corrosion protection for coils during storage and transport. These are straight oils with limited lubrication ability. Many steel companies producing galvanneal coatings have the capability of also applying a phosphate conversion coating called “prephos.” Parts may be stamped with the application of mill oil over this pretreatment, with or without additional press applied lubricant.
- Prelube: These are higher viscosity straight oils or dry barrier lubricant applied at the sheet mill or processor, providing corrosion protection for coils during storage and transport, but with much higher lubricity than mill oils. These can often be used in forming operations without the need for additional press applied lubricant. Some mill oils and liquid prelubes are designed to minimize oil migration in coils during storage. Dry barrier lubricants eliminate migration and provide consistent, low friction.
- Blankwash: These may be preformed emulsions, or very low viscosity straight oils. Their use is typically found on class A parts, applied immediately prior to stamping to remove dirt and metal slivers to prevent surface defects. Although not considered heavy duty lubricants, they contribute to stamping performance.
Press applied metalforming lubricants: These are applied at the press and may be straight oils, heavy duty emulsifiable oils or pre-formed emulsions, or water-based synthetic formulations depending on the severity of the operation and materials being formed. This type of lubricant is most likely to contain EP lubricant additives.
Figure 4 compares important capabilities of some of the types of metalforming lubricants used for forming HSS (after Citation B-35). Prelubes are not shown specifically, but they are similar to mill oil in most respects, but with much improved lubricity. Prelubes are meant to replace press applied lubricants. This figure ranks typical performance levels, as there can be a range of capabilities for each product type. Consult your lubricant supplier(s) for specific information on their products.
![Figure 4: Comparison of important characteristics associated with different lubricant types, where higher values are better [After Citation B-34].](https://ahssinsights.org/wp-content/uploads/2021/02/2.11.5-Fig4-SpiderGraph.svg)
Figure 4: Comparison of important characteristics associated with different lubricant types, where higher values are better (After Citation B-35).
Mill applied lubricants, including dry barrier lubricants, mill oils, and prelubes, are typically applied electrostatically, resulting in thin (approximately 1.0-1.5 g/m2) uniform coatings prior to re-coiling. However, liquid lubricants may migrate within stored coils resulting in areas of thicker and thinner lubricant films. This can affect friction, resulting in a less robust stamping process. Research has shown that electrogalvanized sheet is more sensitive to lubricant chemistry, while galvanneal is sensitive to the lubricant film thickness.D-24 Several commercial oil film thickness measurement instruments are available from various sources.
Several methods and equipment are capable of coating sheets with press applied lubricants.B-36 Manual application with rollers, brushes, and swabs requires little expense, but is generally slow and presents housekeeping challenges. Roller coaters and spray systems are more frequently used. Roller coaters are limited by blank size and steel must be flat. Spray systems can accommodate lubrication in multiple stations, useful for cooling, and is not part shape or size limited. High viscosity lubricants may be difficult to spray. Flood systems offer excellent cooling. Blankwash systems are recirculated flood applications. These must be monitored for changes with use as recommended by the suppler, including contamination, microbiology for water-based lubricants, and viscosity for oil based lubricants.
Iron is available on the surfaces of bare steel and galvanneal to react with conventional EP additives bearing sulfur, phosphorus, and chlorine to form effective tribofilms. However, pure zinc hot dipped and electrogalvanized surfaces show only moderate response to additives.S-70 Galvanneal and electrogalvanized microscopic surface features help retain lubricant for the metalforming operation.S-70 Several sources suggest that the coefficient of friction on hot dipped galvanized surfaces vary less than that seen on electrogalvanized surfaces.
Table I presents guidelines for lubricant selection when forming AHSS.B-37
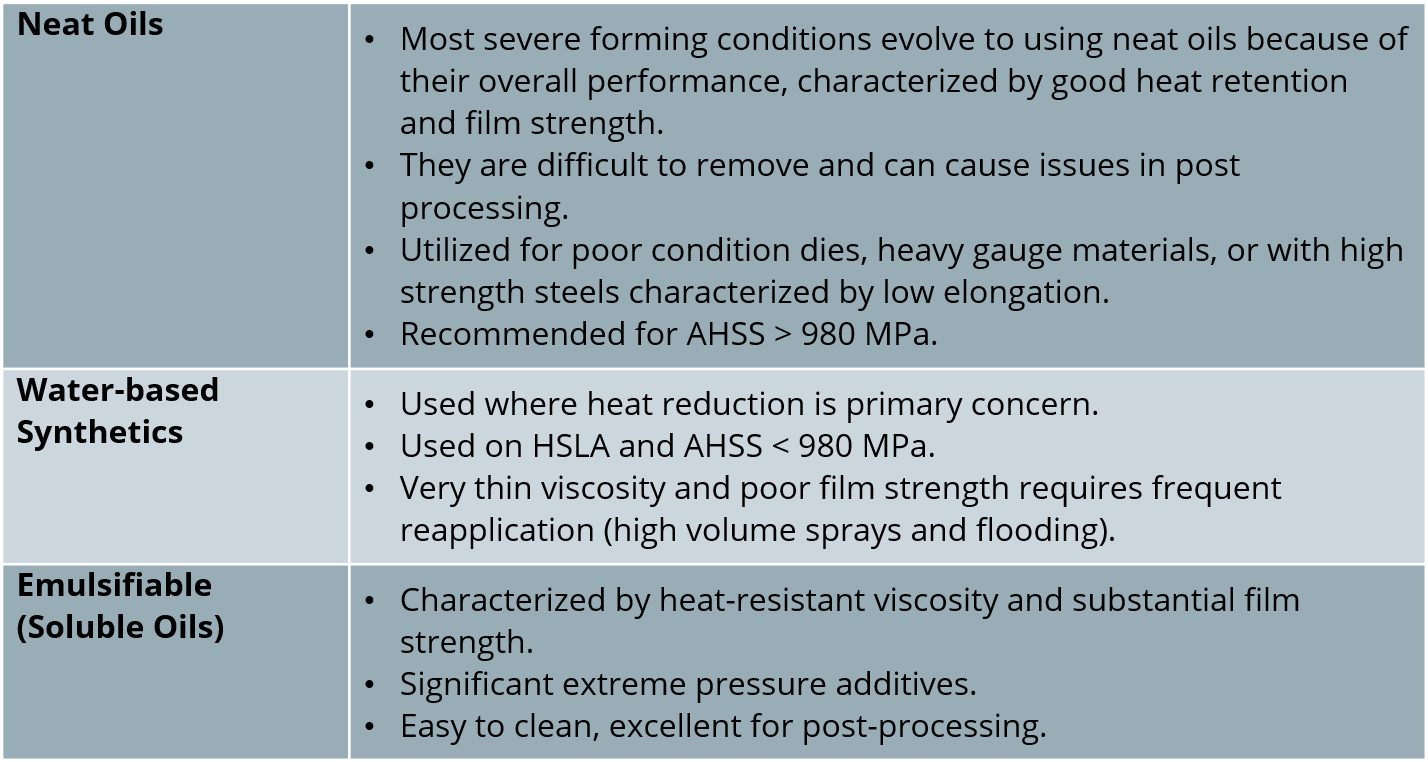
Table I: Lubricant Selection Guide for Forming of AHSS.B-37
Metalforming lubricant manufacturers and end-users conduct many different friction tests to screen lubricants prior to plant trials and to collect friction data for use in modelling. The questions of which test(s) are most applicable to specific operations, and what testing parameters are appropriate for testing, are important considerations. Tribosystem analysis (TSA) is a systematic method that may be applied to a metalforming operation, as well as laboratory tests being considered for use. These analyses are compared in order to determine which laboratory tribotest may be most applicable.S-71 Matching parameters, including test materials, surface features, contact type, and mode of failure are some of the most basic considerations.
Laboratory tribotests have been divided into two main categories: simulation and bench tests. Simulation tests take a metalforming process and scales it down for laboratory use. They are used to study the influence of variables on production, including lubrication. Examples include draw bead simulator (DBS) and cup draw tests (CDT). Bench tests create specific tribological conditions and are used to understand basic phenomena, but may also be applied to results in production, if both the laboratory and production lubrication and wear mechanisms are understood. Examples of bench tests include bending under tension (BUT) and twist compression tests (TCT).S-72
FEM programs have used a single coefficient of friction value in modelling. The coefficient of friction is not uniform across all areas of a part during forming. Programs have been developed that include sheet material and surface properties, lubricant parameters, and tool information to supply FEM programs, friction models for improved accuracy.
High die and part temperatures reached in HSS forming operations puts additional strains on lubricants. EP lubricants are often required at these elevated temperatures. This is particularly true in situations prone to buckling, which creates areas of very high contact pressures, potentially leading to galling. Straight oil viscosity decreases with increasing temperature. The amount of viscosity decrease between 40 °C and 100 °C is reflected in the oil viscosity index (VI).A-55 Reduced oil viscosity can affect lubrication properties.
Some lubricants may emit smoke and odors that must be controlled or eliminated in the workplace. Within base oil types, smoke and flash points increase with viscosity. For a given viscosity, naphthenic base oils have lower smoke and flash points than API Group I, II, III, and IV base oils. Synthetic esters and vegetable oils generally have higher smoke and flash points than corresponding viscosity petroleum oils. Higher temperatures also can increase the oxidation of lubricant residues on parts, forming acidic and polymeric residues, making the lubricants more difficult to remove prior to finishing and potentially reducing interim corrosion protection properties. Antioxidants are often used in lubricants to slow oxidation for this reason. Polymeric oxidation products on steel surfaces, due to high temperatures, may also interfere with welding, adhesive bonding, and coating.
Associations and OEMs have established test programs for testing sheet metal forming lubricants for corrosion protection, cleanability, phosphate application, and coating, as well as adhesive, sealer, and welding compatibility.A-56 Generally, aging of the lubricant films is done before running these tests to simulate time in storage. Higher forming temperatures for HSS can potentially influence performance of lubricants for all these parameters. Tier suppliers within the automotive supply chain are encouraged to use only those lubricants on the OEM approved supplier list.
Sustainability in automobile manufacturing is gaining importanceE-6, which impacts the selection of materials, including metalforming lubricants. Verband Der Automobilindustrie (VDA) published their recommendationsE-4 for guiding principles in the automotive industry for improving sustainability in the supply chain. This includes questionnaires for suppliers and subsuppliers.
Key Points
- Lubrication helps control metal flow from the binder towards the die radius and into the part. Because many high strength parts have less stretch over the punch, different lubricant characteristics must enable additional metal flow in the binder.
- Increased metal strength and reduced sheet thickness for weight reduction require greater press energy and hold down forces. The increased energy to form many AHSS causes both part and die to increase in temperature. Increased temperature usually results in reduced lubricant viscosity and even lubricant breakdown increasing the risk of galling and scoring.
- Maintaining metal flow in the binder requires a robust lubricant with a lower coefficient of friction and resistance to temperature degradation.
- The dry barrier lubricants provide uniform, low friction coatings for drawing AHSS parts.
- EP lubricants can help prevent galling under high temperatures reached when forming AHSS.
- A metalforming operation should be viewed as a system when selecting lubricants, including all relevant mechanical, chemical, and environmental factors. These include the materials and surface properties, contact geometry, loading, motion, and environment.
- Liquid metalforming lubricants may be classified by the amount of mineral oil contained. This influences lubrication, application method, cooling, corrosion protection, and removability. Lubricants may be further classified according to how they are used.
- Software programs have been developed that include sheet material and surface properties, lubricant parameters, and tool information which serve as inputs to FEM programs and friction models, further improving accuracy.
- High forming temperatures of AHSS reduces the viscosity of oils, affecting friction. High temperatures can also cause lubricants to oxidize, fume and smoke, and influence corrosion protection, removability, welding, adhesive bonding, and coating.
- Sustainability is becoming a lubricant selection criterion.
Back To Top
Blog, homepage-featured-top, News
We thank Dennis McPike, Zapp Tooling Alloys, Inc. for contributing this insightful case study.
Multi-phase steels are complex to cut and form, requiring specific tooling materials. The tooling alloys which have been used for decades, such as D2, A2 or S7, are reaching their load limits and often result in unacceptable tool life. The mechanical properties of the sheet steels achieve tensile strengths of up to 1800 MPa with elongations of up to 40%. Additionally, the tooling alloys are stressed by the work hardening of the material during processing.
The challenge to process AHSS quickly and economically makes it necessary for suppliers to manufacture tooling with an optimal tool steel selection. The following case study illustrates the tooling challenges caused by AHSS and the importance of proper tool steel selection.
A manufacturer of control arms changed production material from a conventional steel to an Advanced High-Strength Steel (AHSS), HR440Y580T-FB, a Ferrite-Bainite grade with a minimum yield strength of 440 MPa and a minimum tensile strength of 580 MPa. However, the tool steels were not also changed to address the increased demands of AHSS, resulting in unacceptable tool life and down time.
According to the certified metal properties, the 4 mm thick FB 600 material introduced into production had a 525 MPa yield strength, 605 MPa tensile strength, and a 20% total elongation. These mechanical properties did not appear to be a significant challenge for the tool steels specified in the existing die standards. But the problems encountered in production revealed serious tool life problems.
To form the FB 600 the manufacturer used D2 steel. D2 was successful for decades in forming applications. This cold work tool steel is used in a wide variety of applications due to its simple heat treatment and its easily adjustable hardness values. In this case, D2 was used at a hardness of RC 58/60.
While tools manufactured from D2 can withstand up to 50,000 load cycles when forming conventional steels, these particular D2 tools failed after only 5,000 – 7,000 cycles during the forming of FB 600. The first problems were detected on a curl station where mechanical overload caused the D2 tools to break catastrophically, as seen in Figure 1 below. Since the breakage was sudden and unforeseeable, each failure of the tools resulted in long changeover times and thus machine downtime.

Figure 9: Breakage seen in control arm curl tool made from D2, leading to premature failure. Conversion to a PM tool steel having higher impact resistance led to 10x increase in tool life.M-20
Since the cause of failure was a mechanical breakage of the tools, a tougher alternative was consequently sought. These alternatives, which included A2 and DC53 were tested at RC 58-60 and unfortunately showed similar tool life and failures.
Metallurgical analysis indicated that the failure resulted from insufficient impact strength of the tool steel. This was caused by the increased cross-cut that the work-hardened AHSS exerted on the curl. As an alternative material, a cold work steel with a hardness of 58-60, a tensile strength of about 2200 – 2400 MPa and high toughness was sought. These properties could not be achieved with conventional tool steels. The toolmaker used a special particle metallurgy (PM) tool steel to obtain an optimum combination of impact strength, hardness and wear resistance.
Particle metallurgy (PM) tool steels, due to their unique manufacturing process, represent improvements in alloy composition beyond the capabilities of conventional tool steels. Materials with a high alloy content of carbide formers such as chromium, vanadium, molybdenum and tungsten are readily available. The PM melting process ensures that the carbides are especially fine in particle size and evenly distributed (reference Table 1). This process results in a far tougher tool steel compared to conventional melting practices.

Table 1: Elemental Composition of Chosen Tool Steel
The manufacturer selected Z-Tuff PM® to be used at a hardness of RC 58-60. Employing the identical hardness as the conventional cold work steel D2, a significant increase in impact strength (nearly 10X increase as measured by un-notched Charpy impact values) was realized due to the homogeneous microstructure and the more evenly distributed precipitates. This positive effect of the PM material led to a significant increase in tool life. By switching to the PM tool steel, the service life is again at the usual 40000 – 50000 load cycles. By using a steel with an optimal combination of properties, the manufacturer eliminated the tool breakage without introducing new problems such as deformation, galling, or premature wear.
AHSS creates tooling demands that challenge the mechanical properties of conventional tool steels. Existing die standards may not be sufficient to achieve consistent and reliable performance for forming, trimming and piercing AHSS. Proper tool steel grade selection is critical to ensuring consistent and reliable tooling performance in AHSS applications. Powder metallurgical tool steels offer a solution for the challenges of AHSS.