Blog, homepage-featured-top, main-blog
Equipment, Responsibilities, and Property Development Considerations When Deciding How A Part Gets Formed
Automakers contemplating whether a part is cold stamped or hot formed must consider numerous ramifications impacting multiple departments. Over a series of blogs, we’ll cover some of the considerations that must enter the discussion.
The discussions relative to cold stamping are applicable to any forming operation occurring at room temperature such as roll forming, hydroforming, or conventional stamping. Similarly, hot stamping refers to any set of operations using Press Hardening Steels (or Press Quenched Steels), including those that are roll formed or fluid-formed.
Equipment
There is a well-established infrastructure for cold stamping. New grades benefit from servo presses, especially for those grades where press force and press energy must be considered. Larger press beds may be necessary to accommodate larger parts. As long as these factors are considered, the existing infrastructure is likely sufficient.
Progressive-die presses have tonnage ratings commonly in the range of 630 to 1250 tons at relatively high stroke rates. Transfer presses, typically ranging from 800 to 2500 tons, operate at relatively lower stroke rates. Power requirements can vary between 75 kW (630 tons) to 350 kW (2500 tons). Recent transfer press installations of approximately 3000 tons capacity allow for processing of an expanded range of higher strength steels.
Hot stamping requires a high-tonnage servo-driven press (approximately 1000 ton force capacity) with a 3 meter by 2 meter bolster, fed by either a roller-hearth furnace more than 30 m long or a multi-chamber furnace. Press hardened steels need to be heated to 900 °C for full austenitization in order to achieve a uniform consistent phase, and this contributes to energy requirements often exceeding 2 MW.
Integrating multiple functions into fewer parts leads to part consolidation. Accommodating large laser-welded parts such as combined front and rear door rings expands the need for even wider furnaces, higher-tonnage presses, and larger bolster dimensions.
Blanking of coils used in the PHS process occurs before the hardening step, so forces are low. Post-hardening trimming usually requires laser cutting, or possibly mechanical cutting if some processing was done to soften the areas of interest.
That contrasts with the blanking and trimming of high strength cold-forming grades. Except for the highest strength cold forming grades, both blanking and trimming tonnage requirements are sufficiently low that conventional mechanical cutting is used on the vast majority of parts. Cut edge quality and uniformity greatly impact the edge stretchability that may lead to unexpected fracture.
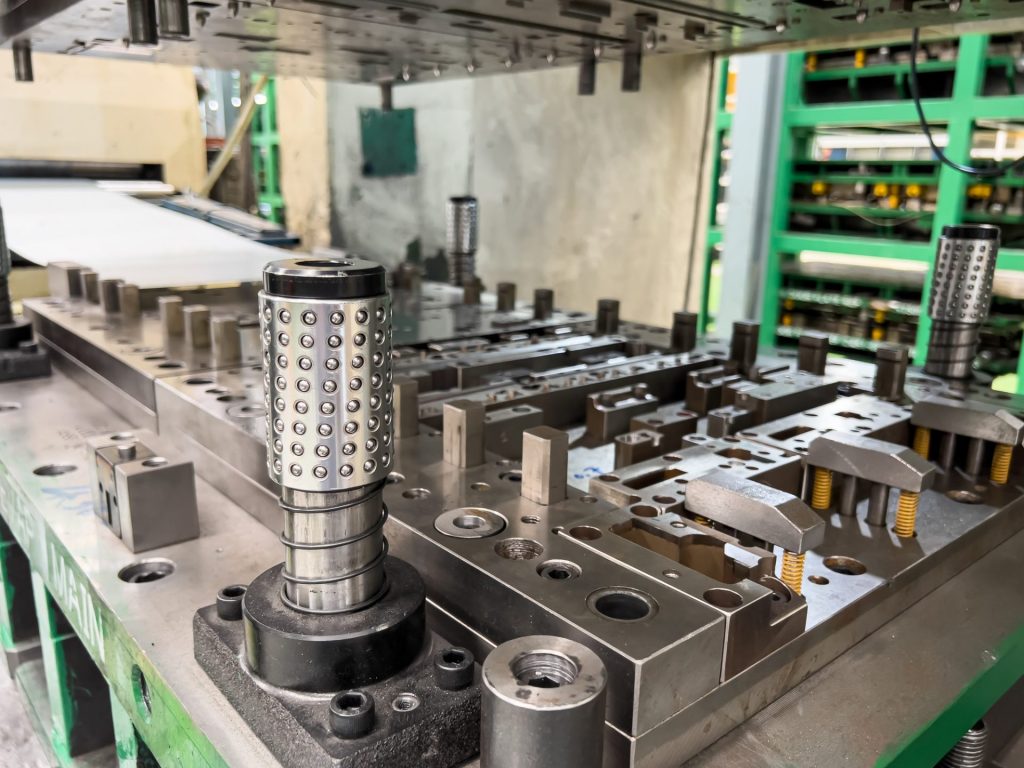
Responsibilities
Most cold stamped parts going into a given body-in-white are formed by a tier supplier. In contrast, some automakers create the vast majority of their hot stamped parts in-house, while others rely on their tier suppliers to provide hot stamped components. The number of qualified suppliers capable of producing hot stamped parts is markedly smaller than the number of cold stamping part suppliers.
Hot stamping is more complex than just adding heat to a cold stamping process. Suppliers of cold stamped parts are responsible for forming a dimensionally accurate part, assuming the steel supplier provides sheet metal with the required tensile properties achieved with a targeted microstructure.
Suppliers of hot stamped parts are also responsible for producing a dimensionally accurate part, but have additional responsibility for developing the microstructure and tensile properties of that part from a general steel chemistry typically described as 22MnB5.
Property Development
Independent of which company creates the hot formed part, appropriate quality assurance practices must be in place. With cold stamped parts, steel is produced to meet the minimum requirements for that grade, so routine property testing of the formed part is usually not performed. This is in contrast to hot stamped parts, where the local quench rate has a direct effect on tensile properties after forming. If any portion of the part is not quenched faster than the critical cooling rate, the targeted mechanical properties will not be met and part performance can be compromised. Many companies have a standard practice of testing multiple areas on samples pulled every run. It’s critical that these tested areas are representative of the entire part. For example, on the top of a hat-section profile where there is good contact between the punch and cavity, heat extraction is likely uniform and consistent. However, on the vertical sidewalls, getting sufficient contact between the sheet metal and the tooling is more challenging. As a result, the reduced heat extraction may limit the strengthening effect due to an insufficient quench rate.
For more information, see our Press Hardened Steel Primer to learn more about PHS grades and processing!
Thanks are given to Eren Billur, Ph.D., Billur MetalForm for his contributions to the Equipment section, as well as many of the webpages relating to Press Hardening Steels at www.AHSSinsights.org.
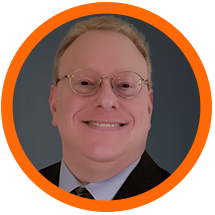
Danny Schaeffler is the Metallurgy and Forming Technical Editor of the AHSS Applications Guidelines available from WorldAutoSteel. He is founder and President of Engineering Quality Solutions (EQS). Danny wrote the monthly “Science of Forming” and “Metal Matters” column for Metalforming Magazine, and provides seminars on sheet metal formability for Auto/Steel Partnership and the Precision Metalforming Association. He has written for Stamping Journal and The Fabricator, and has lectured at FabTech. Danny is passionate about training new and experienced employees at manufacturing companies about how sheet metal properties impact their forming success.
Press Hardened Steels
Overview
The initial press hardening steels of the 1970s were delivered bare, without a galvanized or aluminized layer for corrosion protection (i.e., uncoated). During the heating process, an oxide layer of FeOx forms if the furnace atmosphere is not controlled. Through the years, several coating technologies have been developed to solve the following problems of uncoated steelsF-14, F-33:
- Scale formation, which causes abrasive wear and requires a secondary shotblasting process before welding,
- Decarburization, which leads to softening close to the surface,
- Risk of corrosion.
The first commercially available coating on press hardening steels was patented in 1998. The coating was designed to solve the scaling problem, but it also offered some corrosion resistance.C-24 Since the coating composition is primarily aluminium, with approximately 9% silicon, it is usually referred to as AlSi, Al-Si, or AS.
Coating thickness is nominally 25 μm (75 g/m2) on each side and referenced as AS150. A more recent offering is a thinner coating of 13 μm (AS80).A-51 The AS coating requires a special heating curve and soaking time for better weldability, corrosion resistance and running health of the furnace. Most OEMs now include furnace dew point limitations to reduce/avoid hydrogen embrittlement risk.
In 2005, Volkswagen was looking for a method to manufacture deep drawn transmission tunnels and other complex-to-form underbody components using press hardened steels. Although AS coatings were available, parts could not be formed to the full draw depth using the direct process, and AS coated blanks cracked during the cold forming portion of the two-step hybrid process. Using uncoated blanks led to severe scale formation, which increased the friction coefficient in hot forming. For this particular problem, a varnish coating was developed. The coating was applied at a steel mill, and shipped to Volkswagen’s stamping plant. The parts were first cold pre-formed and then heated in a furnace, as seen in Figure 1a. Hot pre-forms were then deep drawn to tunnels. As shown in Figure 1b, scale formed on parts which did not have the coating. A varnish coated blank could be cold formed without any scale, Figure 1c.S-63, F-34 Since then, some other varnish coatings also have been developed.
![Figure 1: Transmission tunnel of 2005 Volkswagen Passat: (a) hot forming of pre-form, and final parts: (a) uncoated blank would suffer from scaling, (c) scale-free parts can be formed from varnish-coated blanks [REFERENCE 7]](https://ahssinsights.org/wp-content/uploads/2021/02/Fig1_Transmission-tunnel-of-2005-Volkswagen-Passat.jpg)
Figure 1: Transmission tunnel of 2005 Volkswagen Passat: (a) hot forming of pre-form, and final parts: (a) uncoated blank would suffer from scaling, (c) scale-free parts can be formed from varnish-coated blanks.F-34
In car bodies, components that are sealed from external moisture are referred to as dry areas. These areas have low risk of corrosion. Areas that may be exposed to moisture are wet areas. Precautions must be taken to avoid corrosion of the sheet metal, such as using galvanized or pre-coated steel. Sealants can also be applied to joints to keep out moisture. The presence of humidity in these areas increases the risk of forming a galvanic cell, leading to accelerated corrosion. These areas have higher risk of corrosion and may require additional measures. Figure 2 shows dry and wet areas. In this figure, parts colored with yellow may be classified as wet or dry, depending on the vehicle design and the OEMs requirements.G-41
![Figure 2: Dry and wet areas in a car body. [REFERENCE 8]](https://ahssinsights.org/wp-content/uploads/2021/02/Fig2_Dry-and-wet-areas-in-a-car-body.jpg)
Figure 2: Dry and wet areas in a car body.G-41
An estimated ~40% of press hardened components are in dry areas. Thus, high corrosion protection is desired in the 60% of all press hardened components which are employed in wet areas.B-48 Zn-based coatings are favored for their cathodic protection, but require tight process control. The first commercial use of Zn-coated PHS was in 2008, using the indirect process.P-20 Since then, direct forming of Zn-coated PHS has been studied. When direct formed, furnace soaking temperature and time must be controlled carefully to avoid deep microcracks.G-41, K-20 Recently developed are two new Zn-coated press hardening steel grades, 20MnB8 and 22MnSiB9-5, both reaching approximately 1500 MPa tensile strength after processing. Using grades requires a pre-cooling process after the furnace to solidify the Zn-based coating. 20MnB8 can be direct hot formed to final shape, whereas 22MnSiB9-5 can be formed in a transfer press in the “multi-step” process.K-21, H-27
Depending on the coating type and thickness, the process type, controls and investment requirements may change significantly. For example, some press hardening lines may be designed to form blanks with only Al-based coatings. Table 1 summarizes the advantages and disadvantages of several coating systems.
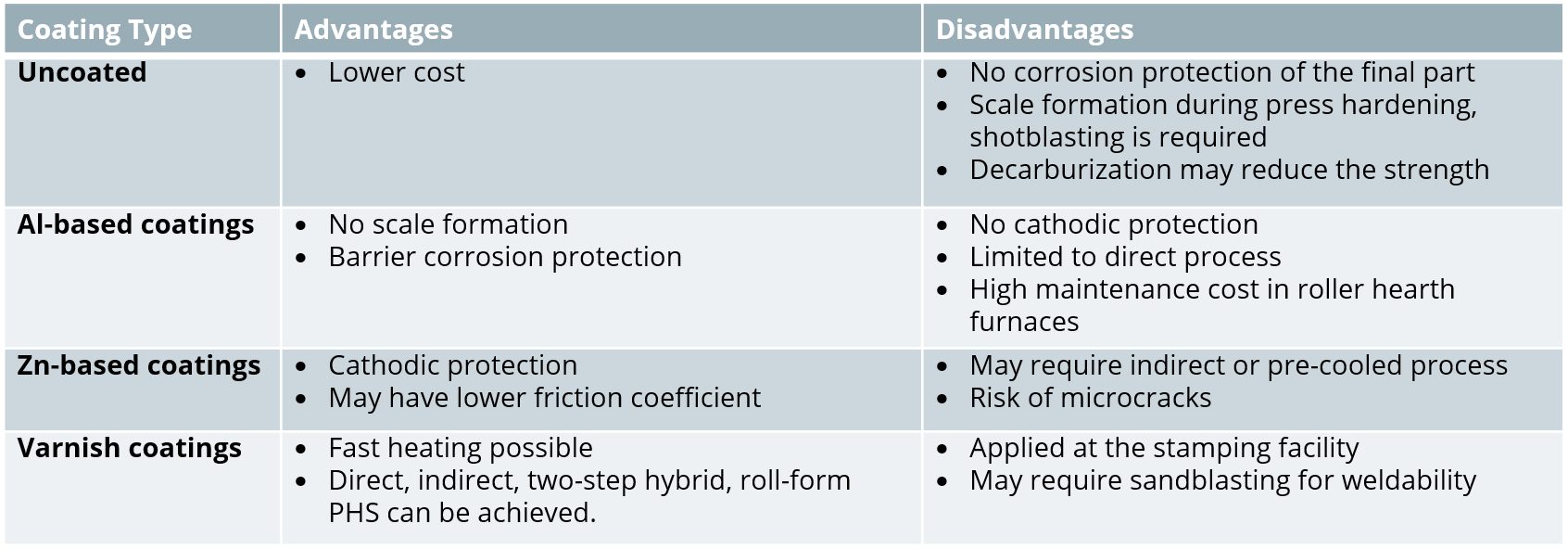
Table 1: Summary of coatings available for press hardening steels.
Uncoated Blanks
The earliest press hardening steels did not have any coating on them. These steels are still available and may be preferred for dry areas in automotive applications. If the steel is uncoated and the furnace atmosphere is not controlled, scale formation is unavoidable. Scale is the term for iron oxides which form due to high temperature oxidation. Scale thickness increases as the time in furnace gets longer, as seen in Figure 3. Scale has to be removed before welding, requiring a shotblasting stage. Thicker scale is more difficult and more costly to remove.M-53 Early attempts to reduce (if not avoid) scale formation saw the use of an inert-gas atmosphere inside the furnace.A-52 Today, a mixture of nitrogen (N2) and natural gas (CH4) is typically used.F-35 In China, at least one tier supplier is using a vacuum furnace to prevent scale formation.A-68
![Figure 3: Oxide layer (scale) on press hardened steel after: (a) fast resistance heating (10 seconds in air), (b) furnace heating (120 seconds in air) [REFERENCE 14]](https://ahssinsights.org/wp-content/uploads/2021/02/Fig3_Oxide-layer-on-press-hardened-steel-after-fast-resistance-heating.jpg)
Figure 3: Oxide layer (scale) on press hardened steel after: (a) fast resistance heating (10 seconds in air), (b) furnace heating (120 seconds in air).M-53
While heating uncoated steel in the furnace, if the conditions are favorable for iron (Fe) oxidation, carbon (C) may also be oxidized. When the carbon is oxidized, layers close to the surface lose their carbon content as gaseous carbon monoxide (CO) and/or carbon dioxide (CO2) is produced.S-87 The depth of the “decarburization layer” increases with dwell time in the furnace, until an oxide layer (scale) formed. Scale acts as a barrier between the bare steel and atmosphere. As the carbon is depleted in the “decarburization layer”, the hardness of the layer is decreased, as seen in Figure 4. Decarburization is usually undesirable since it lowers the strength/hardness and may negatively affect fatigue life.C-26

Figure 4: Hardness distribution of an uncoated steel after 6 minutes in a 900 °C furnace, showing hardness decrease as the surface layers lose their carbon. Image recreated after C-26.
Several methods are available to improve the corrosion resistance of uncoated PHS parts:
- E-coating after welding, before painting is a typical step of car body manufacturing, for rustproofing.
- If descaling can be done by using chromium shots (in shotblasting), a thin film of chromium-iron may grow on the surface and improve the corrosion resistance.F-14
- Vapor galvanizing (also known as Sherardizing) of uncoated steel after descaling, an experimental study described in Citation G-42.
- Electro-galvanizing after hot stamping, as described in Citation A-68.
- Change the base metal chemistry to one that is more oxidation resistant.L-60 Figure 5 compares the shiny non-oxidized surface appearance of parts made from this grade with that made from a conventional uncoated press hardening grade on the same production line with the same processing conditions.W-28

Figure 5: Oxidation resistant PHS grades may not need descaling or coatings for sufficient corrosion resistance.W-28
Aluminium-Based Coatings
The first commercially available coating on press hardening steels was patented by Sollac (now part of ArcelorMittal) in 1998. This coating was designed to address the scaling problem, but also offers some barrier corrosion resistance.C-24 The nominal coating composition is 9-10 wt.% Si, 2-4 wt.% Fe, with the balance Al.L-39 The coating may be referred to as AlSi, Al-Si, AluSi or more commonly AS. Nominal as-delivered coating thickness is 25 μm (approximately 75 g/m2) on each side, and is usually referred to as AS150, with 150 referencing the total coating weight combining both sides, expressed as g/m2. More recently, a thinner coating of 13 μm (30-40 g/m2 on each side, AS60 or AS80) is now commercially available.A-51 When AS coated blanks are “tailor rolled,” the coating thickness is also reduced in a similar percentage of the base metal thickness reduction. Corrosion protection is similarly reduced, and furnace parameters need to be adjusted accordingly.
As delivered, AS150 has a coating thickness of 20-33 μm and a hardness of approximately 60 HV. The “interdiffusion layer” (abbreviated as IDL) has a high hardness and low toughness at delivery, as seen in Figure 6a. Due to the brittle nature of the IDL, AS coated blanks cannot be cold formed unless very special precautions are taken. During heating, iron from the base metal diffuses to the coating forming very hard AlSiFe (or AlFe) layers close to surface. At the same time, Al and Si of the coating diffuse to the IDL, growing it in thickness and reducing its hardness, Figure 6b. Earlier studies have shown that heating time (and also furnace temperature) has direct effect on the final thickness of IDL, as shown in Figure 7. Once the IDL thickness surpasses approximately 16 to 17 μm, the welding current range (ΔI = Iexpulsion – Imin) may be well below 2 kA.V-15, V-21, W-34 The dwell time must be long enough to ensure proper surface roughness (see Figure 6b) for e-coatability.M-27, T-40 Figure 10 summarizes the heating process window of AS coatings. The process window may change with base metal and coating thicknesses.

Figure 6: AS coating micrographs: (a) as-delivered, (b) after hot stamping process (re-created after V-15, V-21, W-34, G-32)
![Figure 6: IDL thickness variation with furnace dwell time (Image created by REFERENCE 43 using raw data from REFERENCE 22, REFERENCE 26, and REFERENCE 27]](https://ahssinsights.org/wp-content/uploads/2021/02/Fig6_IDL-thickness-variation-with-furnace-dwell-time.jpg)
Figure 7: IDL thickness variation with furnace dwell time (Image created by B-55 using raw data from V-21, G-32, K-41.)
Hydrogen induced cracking (HIC, also known as hydrogen embrittlement) has been a major problem for steels over 1500 MPa tensile strength. AS coated steels may have higher diffusible hydrogen, when delivered, due to the aluminizing process occurring at 680 °C. In addition, AS coated grades may have a hydrogen absorption rate up to three times higher during heating.C-27 To reduce the hydrogen diffusion, it is essential to control the heating process (both heating rate and dew point in the furnace). AS coated blanks absorb hydrogen at room temperature; however, this happens at much lower rates than uncoated or Zn-coated blanks.J-21 Diffusible hydrogen can be removed from the press hardened part by re-heating the part to around 200 °C for 20 minutes or longer, in a process called de-embrittlement.V-21, G-32, G-43, J-21
For the abovementioned reasons, AS coated higher strength grades (i.e., PHS1800 and over) are required to have precise “dew point regulations” during the heating in furnace. Their final properties, especially elongation and bending angle, may be guaranteed only after bake hardening, as shown in Figure 8.B-32 Paint baking is standardized in Europe as a treatment for 20 minutes at 170 °C, which may act like a de-embrittlement treatment.E-10 Some OEMs also require dew point control and “subsequent de-embrittlement treatment” for AS coated PHS1500.
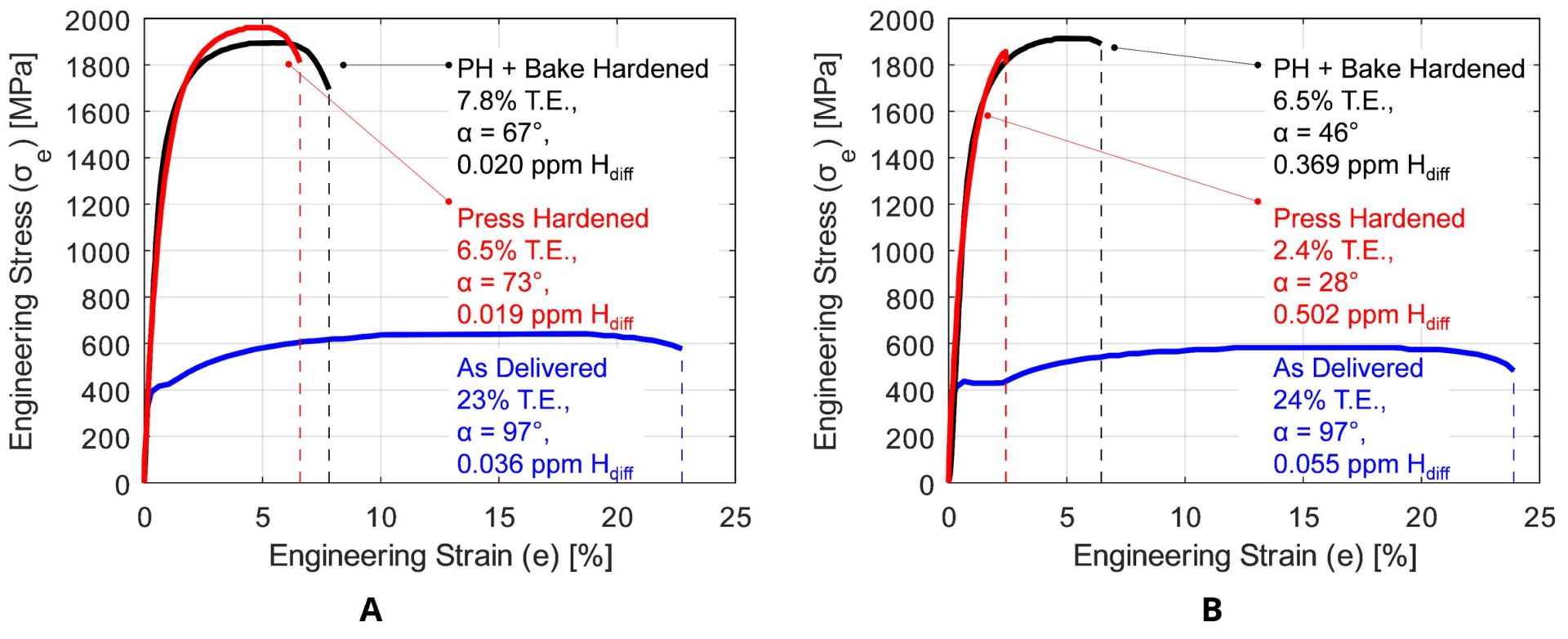
Figure 8: Effect of diffusible hydrogen (Hdiff) on mechanical properties of: (a) uncoated PHS2000, (b) AS coated PHS2000 in an uncontrolled furnace atmosphere (B-55 using raw data from C-27).
Another method to reduce the risk of hydrogen embrittlement is to adjust the coating composition. The bath chemistry for a standard AlSi coating consists of up to 90% aluminum, about 8% to 11% silicon and a maximum of 4% iron. Adding a maximum of 0.5% alkaline earth metals, like magnesium, for example has been shown to result in 40% less hydrogen diffusion into steel.R-29, T-45
Although not common in the industry, Al-Zn and Zn-Al-Mg based coatings have also been developed for press hardening processes.F-14 Recently introduced is an aluminium-silicon coating with magnesium additions. When oxidized with water vapor, Mg releases less H2 and thus may reduce the diffusible hydrogen.S-88
AS coatings may cause costly maintenance issues in roller hearth furnaces, as the coating may contaminate the rollers.B-14 Special care has to be taken to avoid the issue or prolong the maintenance intervals.
Zinc-Based Coatings
AS coatings provide some corrosion protection, known as “barrier protection”, as the coating forms a barrier between the oxidizing environment and the bare steel. It is quite common in Europe for a car to have 12 years corrosion protection warranty. To achieve such corrosion resistance, a typical car may have over 85% of its components galvanized.S-89
The use of Zn-coated PHS has been relatively low, compared to AS coated and uncoated grades. In 2015, 76% of the PHS sold in EU27+Turkey was AlSi coated. In these markets, 18% of the PHS sold was uncoated and only 6% was Zn coated.D-20 This can be attributed to the susceptibility of Zn-coated PHS to Liquid Metal Embrittlement (LME, also known as Liquid Metal Assisted Cracks (LMAC) and Liquid Metal Induced Embrittlement (LMIE)).C-28, L-46
After heating and soaking in the furnace, the base metal should be in the austenitic phase. During heating, the Zn coating reacts with the base metal and forms a thin solid layer of body-centered-cubic solid solution of Zn in α-Fe, shown as α-Fe(Zn) in Figure 9. During deformation, a microcrack can be initiated in this layer at the grain boundaries of the austenite in the base metal, as indicated in Figure 9a. As the crack propagates, zinc from the α-Fe(Zn) layer diffuses along the austenite grain boundary and combines with iron from the base steel to form additional α-Fe(Zn), Figure 9b. Cracks propagate through the weak a-Fe(Zn) grain boundary layer, allowing liquid zinc (with diffused iron) to advance into the capillary crack (Figure 9c). After quenching, the base metal transforms to martensite and the liquid Zn transforms to a hard and brittle intermetallic phase, Γ-Fe3Zn10.C-28
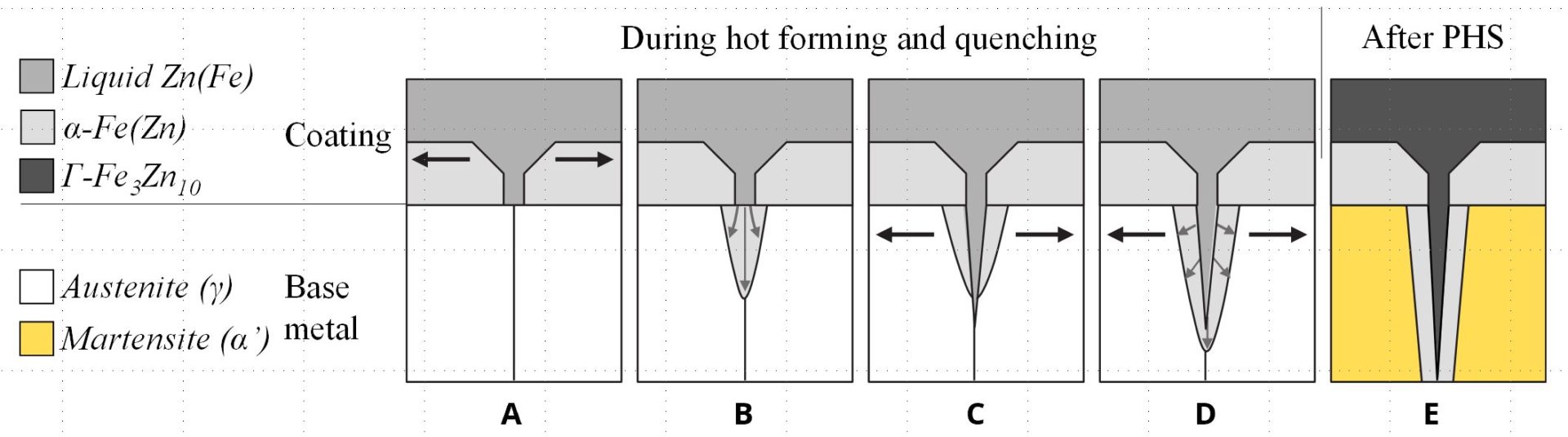
Figure 9: Schematic illustration of microcrack formation. (re-created based on C-28.)
To avoid LME, three methods can be employedK-20:
- Forming in the absence of liquid Zn,
- Reducing stress level,
- Reducing material susceptibility.
There are no breakthroughs to address the last two items. Forming a part in the absence of liquid Zn involves either of two process routes: (1) Indirect press hardening (also known as form hardening), or (2) Pre-cooled direct processes.
In the direct forming of Zn-coated blanks, with or without pre-cooling, microcracks in the base metal may be observed. Microcracks less than 10 μm into the base metal does not affect the fatigue strength of the part.K-20 Microcrack depth is a function of coating thickness, furnace conditions (temperature and dwell time, see Figure 10), forming severity and forming temperature. It may be possible to direct form galvannealed (GA coated) blanks.
The boiling point of pure zinc (907 °C) is very close to the austenitization temperature of 22MnB5 (885 °C), so the heating process window of Zn-coated blanks must be controlled precisely. When the furnace dwell time is too short, deeper microcracks may be observed. When the furnace dwell time is too long, corrosion performance may be degraded. Thus, heating process window of Zn-coated blanks is significantly narrower than that of AS-coated blanks.B-14, S-90
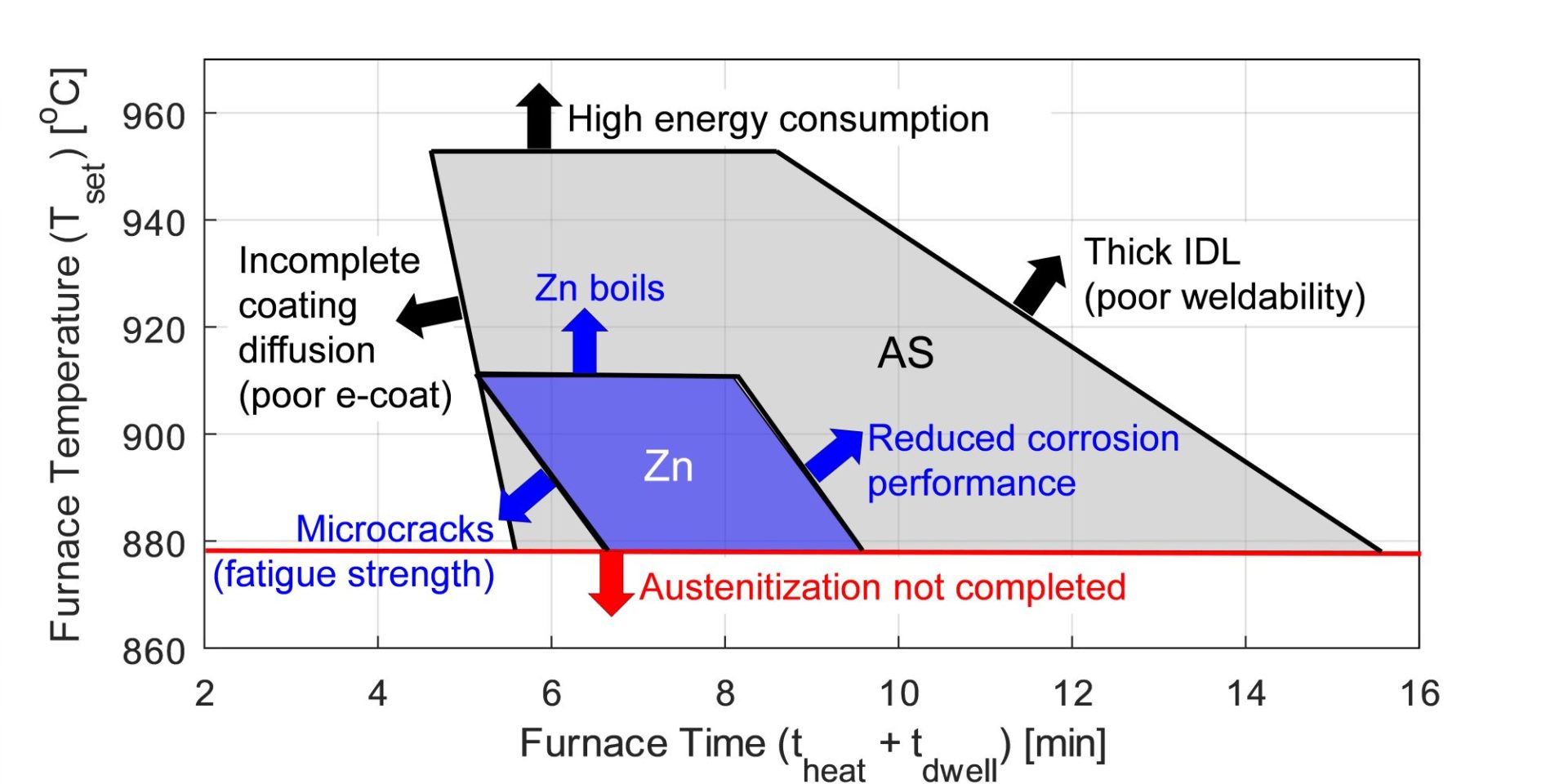
Figure 10: Heating process window of AS and Zn coatings (representative data, may not be accurate for all sheet and coating thicknesses, re-created based on B-14, S-90.
Zn-based coatings may result in very low diffusible hydrogen after press hardening. In one studyJ-21, no diffusible hydrogen was detected, as long as the furnace dwell times are shorter than 6 minutes. Even after 50 minutes in the furnace, diffusible hydrogen was found to be around 0.06 ppm. Zn coatings do not act as a barrier for hydrogen desorption (losing H through the surface). Even at room temperature, Zn coated blanks may lose most of the diffusible hydrogen within a few days (also referred to as aging).
![Figure 10: Evolution of galvanized coating: (a) as delivered: Ferrite+Pearlite in base metal, almost pure Zn coating with Al-rich inhibition layer, (b) at high temperatures: austenite in base metal + α-Fe(Zn) and liquid Zn + surface oxides, (c) after press hardening: martensite in base metal + α-Fe(Zn) and Γ-phase coatings + surface oxides. The oxides are removed prior to welding and painting [REFERENCE 30]](https://ahssinsights.org/wp-content/uploads/2021/02/Fig10_Evolution-of-galvanized-coating.jpg)
Figure 11: Evolution of galvanized coating: (a) as delivered: Ferrite+Pearlite in base metal, almost pure Zn coating with Al-rich inhibition layer, (b) at high temperatures: austenite in base metal + α-Fe(Zn) and liquid Zn + surface oxides, (c) after press hardening: martensite in base metal + α-Fe(Zn) and Γ-phase coatings + surface oxides. The oxides are removed prior to welding and painting.J-21
Zn-based coatings may have a yellowish color after hot stamping. The surface oxides have to be removed before welding. This is typically done by shotblasting.
PHS blanks with a ZnNi coating were previously available. The ZnNi coating provided a low friction coefficient, a large process window in the furnace, the ability to be cold formed (indirect or two-step hybrid processes were also possible) and decreased susceptibility to LME.B-56 ZnNi coated PHS was used in the rear rail of the Opel Adam city carH-57 for a short period, until the coating was discontinued.C-29
Varnish Coatings
Another method to avoid scaling and decarburization is to apply varnish coatings. In this method, uncoated steel can be either coil coated or blanks can be manually coated with the paint-like varnish coatings.B-14 These coatings may also be known as “paint-type” or “sol-gel”.
![Figure 11: Manual application of a varnish coating. [REFERENCE 7]](https://ahssinsights.org/wp-content/uploads/2021/02/Fig11_Manual-application-of-a-varnish-coating.jpg)
Figure 12: Manual application of a varnish coating.F-34
Depending on the type of coating, they may allow very fast heating – including induction and conduction heating with electric current. Since the coating does not require time to diffuse, furnace heating may be completed in less than 2 minutes.F-34 Again, depending on the type, surface conditioning may not be required before welding or e-coating.B-14
They were used in automotive industry between 2005 and 2010. By 2015 there were four different types of varnish coatings, some of which are now discontinued.B-14 These coatings may be useful for prototyping and low volume production.
Back To Top
Blog, RSW Modelling and Performance
Modern car bodies today are made of increasing volumes of Advanced High-Strength Steels (AHSS), the superb performance of which facilitates lightweighting concepts (see Figure 1). To join the different parts of a car body and create the crash structure, the components are usually welded to achieve a reliable connection. The most prominent welding process in automotive production is resistance spot welding. It is known for its great robustness, and easily applicable in fully automated production lines.
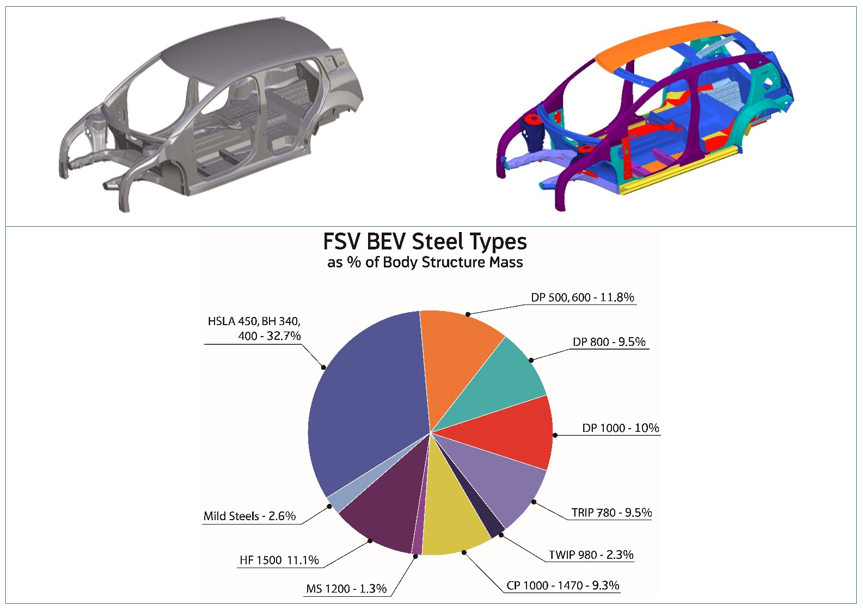
Figure 1: AHSS Content in Modern Car Body.W-7
There are, however, challenges to be met to guarantee a high-quality joint when the boundary conditions change, for example, when new material grades are introduced. Interaction of a liquefied zinc coating and a steel substrate can lead to small surface cracks during resistance spot welding of current AHSS, as shown in Figure 2. This so-called liquid metal embrittlement (LME) cracking is mainly governed by grain boundary penetration with zinc, and tensile stresses. The latter may be induced by various sources during the manufacturing process, especially under ‘rough’ industrial conditions. But currently, there is a lack of knowledge, regarding what is ‘rough’, and what conditions may still be tolerable.
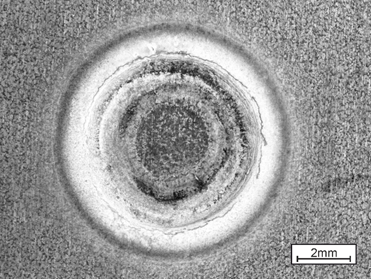
Figure 2: Top View of LME-Afflicted Spot Weld.
The material-specific amount of tensile stresses necessary for LME enforcement can be determined by the experimental procedure ‘welding under external load’. The idea of this method, which is commonly used for comparing cracking susceptibilities of different materials to each other, is to apply increasing levels of tensile stresses to a sample during the welding process and monitor the reaction. Figure 3 shows the corresponding experimental setup.

Figure 3: Welding under external load setup.L-51
However, the known externally applied stresses are not exclusively responsible for LME, but also the welding process itself, which puts both thermally and mechanically induced stresses/strains on the sample. Here, the conventional measuring techniques fail. A numerical reproduction of the experiment grants access to the temperature, stress and strain fields present during the procedure, providing insights on the formation of LME. The electro-thermomechanical simulation model is described in detail in Modelling RSW of AHSS. It is used to simulate the welding under external load procedure (see Figure 4).

Figure 4: Simulation Model of Welding Under External Load.
The videos that can be found in the link above show the corresponding temperature and plastic strain fields. As heat dissipates quickly through the water-cooled electrode, a temperature gradient towards the adjacent areas and a local temperature maximum on the surface forms. The plastic strains accumulate mainly at the electrode indentation area. The simulated strain field shows a local maximum of plastic deformation at the left edge of the electrode indentation, amplified by the externally applied stresses and the boundary conditions implied by the procedure. This area correlates with experimentally observed LME cracking sites and paths as shown in Figure 5.
The simulation shows that significant plastic strains are present during welding. When external stresses (in reality e.g. due to poor part fit-up or distorted parts) contribute to the already high load, LME cracking becomes more likely. The numerical simulation model facilitates the determination of material-specific safety limits regarding LME cracking. Parameter variations and their effects on the LME susceptibility can easily be investigated by use of the model, enabling the user to develop strict processing protocols to reduce the likelihood of LME. Finally, these experimental procedures can be adapted to other high-strength materials, to aid their application understanding and industrial set-up conditions.
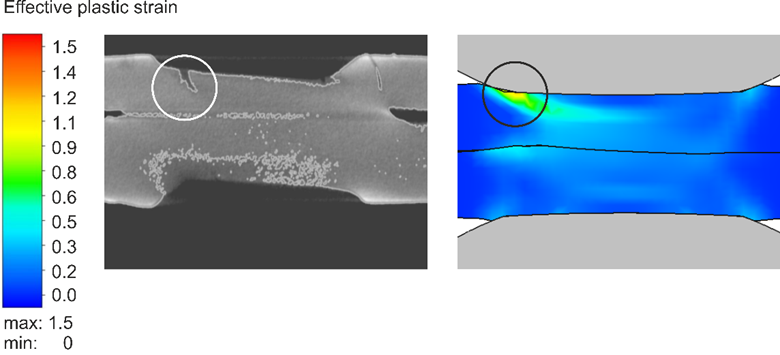
Figure 5: LME Cracks in Cross Section View at Highly Strained Locations.
For more information on this topic, see the paper, co-authored by Fraunhofer and LWF Paderborn, documented in Citation F-23. You may also download the full report documenting the WorldAutoSteel LME project for which this work was conducted.
Blog, main-blog, RSW Modelling and Performance
Modelling resistance spot welding can help to understand the process and drive innovation by asking the right questions and giving new viewpoints outside of limited experimental trials. The models can calculate industrial-scale automotive assemblies and allow visualization of the highly dynamic interplay between mechanical forces, electrical currents and thermal flow during welding. Applications of such models allow efficient weldability tests necessary for new material-thickness combinations, thus well-suited for applications involving Advanced High -Strength Steels (AHSS).
Virtual resistance spot weld tests can narrow down the parameter space and reduce the amount of experiments, material consumed as well as personnel- and machine- time. They can also highlight necessary process modifications, for example the greater electrode force required by AHSS, or the impact of hold times and nugget geometry. Other applications are the evaluation of whole-part distortion to ensure good part-clearance and the investigation of stress, strain and temperature as they occur during welding. This more research-focused application is useful to study phenomena arising around the weld such as the formation of unwanted phases or cracks.
Modern Finite-Element resistance spot welding models account for electric heating, mechanical forces and heat flow into the surrounding part and the electrodes. The video shows the simulated temperature in a cross-section for two 1.5 mm DP1000 sheets:
First, the electrodes close and then heat starts to form due to the electric current flow and agglomerates over time. The dark-red area around the sheet-sheet interface represents the molten zone, where the nugget forms after cooling. While the simulated temperature field looks plausible at first glance, the question is how to make sure that the model calculates the physically correct results. To ensure that the simulation is reliable, the user needs to understand how it works and needs to validate the simulation results against experimental tests. In this text, we will discuss which inputs and tests are needed for a basic resistance spot welding model.
At the base of the simulation stands an electro-thermomechanical resistance spot welding model. Today, there are several Finite Element software producers offering pre-made models that facilitate the input and interpretation of the data. First tests in a new software should be conducted with as many known variables as possible, i.e., a commonly used material, a weld with a lot of experimental data available etc.
As first input, a reliable material data set is required for all involved sheets. The data set must include thermal conductivity and capacity, mechanical properties like Young’s modulus, tensile strength, plastic flow behavior and the thermal expansion coefficient, as well as the electrical conductivity. As the material properties change drastically with temperature, temperature dependent data is necessary at least until 800°C. For more commonly used steels, high quality data sets are usually available in the literature or in software databases. For special materials, values for a different material of the same class can be scaled to the respective strength levels. In any case, a few tests should be conducted to make sure that the given material matches the data set. The next Figure shows an exemplary material data set for a DP1000. Most of the values were measured for a DP600 and scaled, but the changes for the thermal and electrical properties within a material class are usually small.
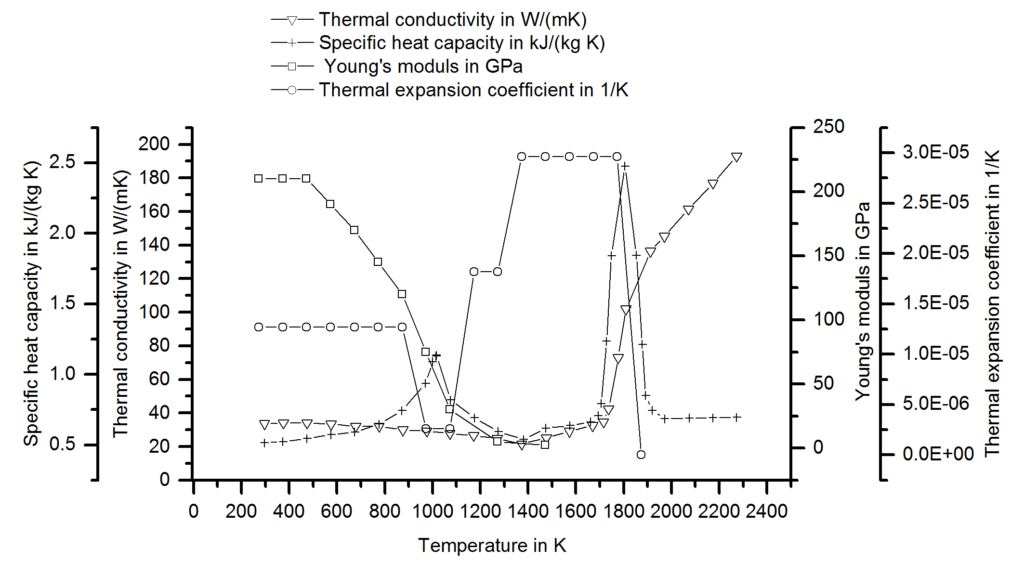
Figure 1: Material Data set for a DP1000.S-73
Next, meaningful boundary conditions must be chosen and validated against experiments. These include both the electrode cooling and the electrical contact resistance. To set up the thermal flow into the electrode, temperature measurements on the surface are common. In the following picture, a measurement with thermocouples during welding and the corresponding result is shown. By adjusting the thermal boundary in the model, the simulated temperatures are adjusted until a good match between simulation and experiment is visible. This calibration needs to be conducted only once when the model is established because the thermal boundary remains relatively constant for different materials and coatings.
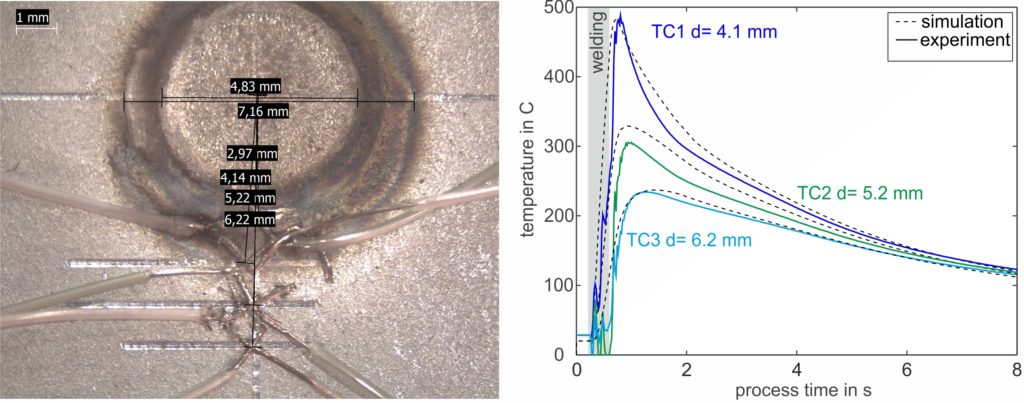
Figure 2: Temperature measurement with thermocouples during welding and the results. The simulated temperature development is compared to the experimental curve and can be adjusted via the boundary conditions.F-23
The second boundary condition is the electrical contact resistance and it is strongly dependent on the coating, the surface quality and the electrode force. It needs to be determined experimentally for every new coating and for as many material thickness combinations as possible. In the measuring protocol, a reference test eliminates the bulk material resistance and allows for the determination of the contact resistances using a µOhm-capable digital multimeter.
Finally, a metallographic cross-section shows whether the nugget size and -shape matches the experiment. The graphic shows a comparison between an actual and simulated cross section with a very small deviation of 0.5 mm in the diameter. As with the temperature measurements, a small deviation is not cause for concern. The experimental measurements also exhibit scatter, and there are a couple of simplifications in the model that will reduce the accuracy but still allow for fast calculation and good evaluation of trends.
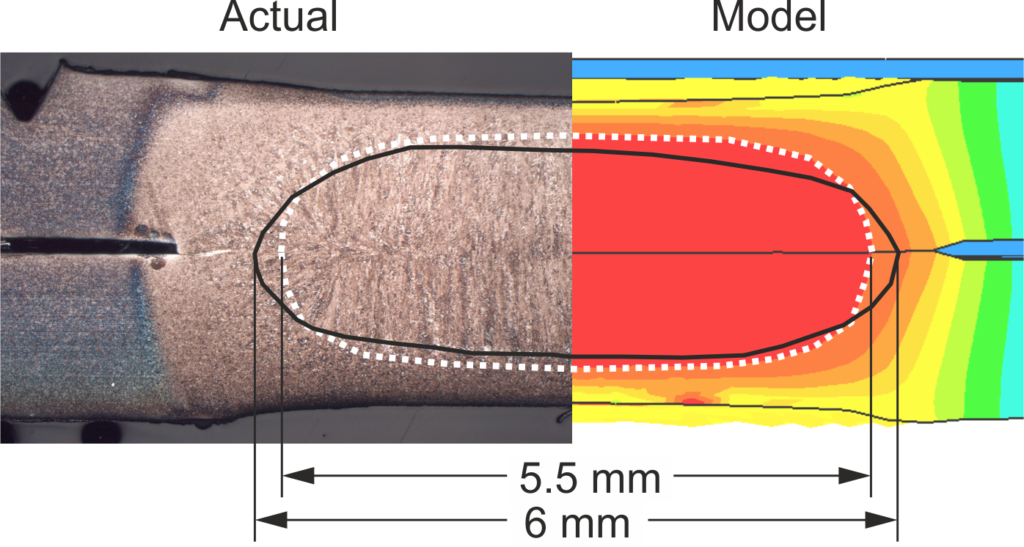
Figure 3: Comparison of experimental and virtual cross-sections.F-23
After validation, consider conducting weldability investigations with the model. Try creating virtual force / current maps and the resulting nugget diameter to generate first guesses for experimental trials. We can also gain a feeling how the quality of each weld is affected by changes in coatings or by heated electrodes when we vary the boundary conditions for contact resistance and electrode cooling. The investigation of large spot-welded assemblies is possible for part fit-up and secondary effects such as shunting. Finally, the in-depth data on temperature flow and mechanical stresses is available for research-oriented investigations, cracking and joint strength impacts.
Note: The work represented in this article is a part a study of Liquid Metal Embrittlement (LME), commissioned by WorldAutoSteel. You can download the free report on the results of the LME study, including how this modelling was used to verify physical tests, from the WorldAutoSteel website.