Testing and Characterization
topofpage
The ISO 16630 Hole Expansion Test and the VDA238-100 bend test are among the few standardized tests to characterize local formability, the term which describes when part and process design, in addition to sheet metal properties like strength and elongation, influence the amount of deformation the metal can undergo prior to failure.
Researchers have developed alternate tests to further investigate process parameters and more clearly understand and optimize non-steel related variables. Also important is the investigation of different strain states from the ones seen in the hole expansion and bending tests. Comparisons of the stretched or bent edge performance evaluating different process parameters using the same material help to better define optimum process parameters. Repeating the testing with different AHSS grades confirms if similar trends exist across different microstructures and strengths. Standardization of these alternate tests has not yet occurred, so use caution when comparing specific values from different studies.
Hole Tension Test
Microstructural damage in the shear affected zone reduces edge ductility. Damage has been evaluated with a modified tensile dogbone containing a central hole prepared by shearing or reaming, as shown in Figure 1. In contrast with a hole expansion test using a conical punch, the researchers described this as a Hole Tension test, which determines failure strain as a function of grade and edge preparation.
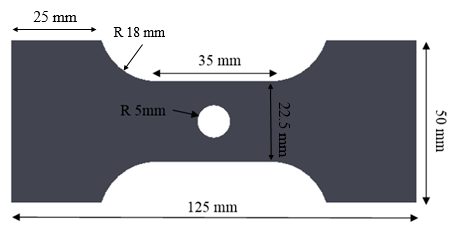
Figure 1: Hole Tension Test Specimen GeometryP-12
Edge Tension Test
A two-dimensional (2-D) edge tension test, also called Half-A-Dogbone test, also evaluates edge stretchability. There are multiple versions of this type of test, but they all are based on the same concept. Like a standard tensile test, the 2-D edge tension test pulls a steel specimen in tension until failure. Unlike a standard tensile test where both sides of the tensile specimen are milled into a “dog bone”, the 2D tension test uses half of a dogbone with different preparation methods for the straight edge and the edge containing the reduced section (Figure 2). The chosen preparation method for each face is a function of the parameter being investigated (ductility, strain, burr, and shear affected zone for example). Potential edge preparation methods include laser cutting, EDM, water jet cutting, milling, slitting or mechanical cutting at various trim clearances, shear angles, rake angles or with different die materials.

Figure 2: 2-D Edge Tension Test Sample. Note the edges are prepared differently based on the targeted property evaluated.
Side Bending Test
Instead of a dogbone or half-dogbone, some studies use a rectangular strip without a reduced section. Bending performance can be evaluated with a rectangular strip having one finished edge and one trimmed edge while preventing out-of-plane buckling, as shown in Figure 3.G-7
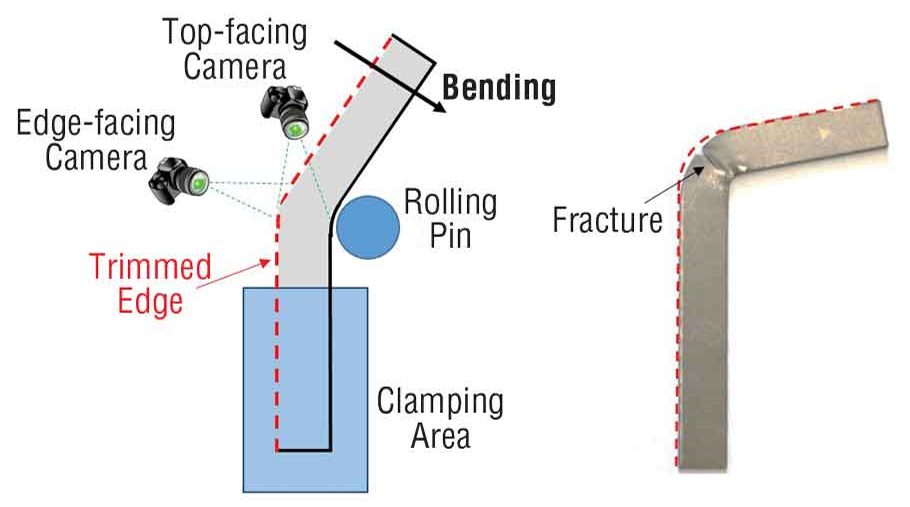
Figure 3: The side-bending test expands a trimmed edge over a rolling pin until detection of the first edge crack.G-7
Half-Specimen Dome Test
Deformation in these three tests occur in the plane of the sheet. Typical hole expansion tests, like production stampings, deform the sheet metal perpendicular to the plane of the sheet. However, hole expansion testing does not always give consistent test results. The half-specimen dome test (HSDT) also attempts to replicate this 3-dimensional forming mode (Figure 4), and appears to be more repeatable likely due to creating a straight cut rather than round hole.
In the HSDT, a rectangular blank is prepared with one edge having the preparation method of interest, like sheared with a certain clearance or laser cut or water-jet cut. The sample is then clamped with the edge to be evaluated over a hemispherical punch. The punch then strains the clamped sample creating a dome shape, with the test stopping with the first crack appears at the edge. Edge stretchability is quantified by measuring dome height or edge thinning or other characteristics.
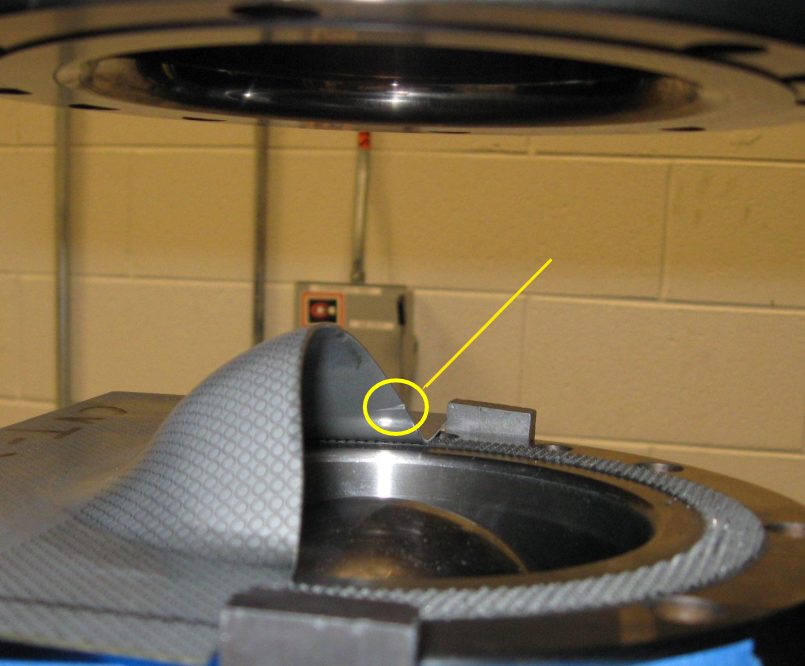
Figure 4: Half-Specimen Dome Test sample. Arrow points to edge crack.S-12
Edge Flange Test
Flanging limits depend on the part contour, edge quality, and material properties. Non-optimal flange lengths – either too long or too short – will lead to fracture. Different tools can assess the influence of flange length, including the one shown in Figure 5 from Citation U-3.

Figure 5: Tool design to investigate flange length before fracture. Flange height: 40mm in left image, 20mm in right image.U-3
Physical tests using this tool show that optimizing sample orientation relative to the rolling direction leads to longer flange lengths before splitting.U-3 Figure 6 highlights the results from testing DP800.

Figure 6: Flange height limits as a function of orientation in DP800.U-3
Back to the Top
Testing and Characterization
The Hole Expansion test (HET) quantifies the edge stretching capability of a sheet metal grade having a specific edge condition. Many variables affect hole expansion performance. By understanding the microstructural basis for this performance, steelmakers have been able to create new grades with better edge stretch capability.
Multiphase microstructures with large hardness differences between the phases, specifically islands of the very hard martensite surrounded by a softer ferrite matrix, may crack along the ferrite-martensite interface (Figure 1). The larger the size of the initiated damage site (due to edge shearing), the smaller the critical stress required for crack propagation.M-6 The microstructure and damage are key components of the Shear Affected Zone, or SAZ.

Figure 1: Features and mechanisms of damage initiation and propagation in Dual Phase steel.M-6
One metallurgical approach to improve sheared edge stretchability is targeting a homogeneous microstructure. Steel suppliers have engineered product offerings like complex phase steel, where extensive grain refinement (reducing the size of the ferrite and martensite grains) is achieved. Consequently, the size of the initial damage resulting from shearing is reduced, raising the critical stress for crack propagation to higher levels and reducing the likelihood for crack propagation. Additionally, reducing the difference in hardness between the soft ferrite phase and the hard martensite phase improves the hole expansion ratio. Changes in chemistry, hot rolling conditions and intercritical annealing temperatures are some of the methods used to achieve this. Such metallurgical trends can include a single phase of bainite or multiple phases including bainite and removal of large particles of martensite. This trend is shown in Figure 2, adapted from Citation M-11.
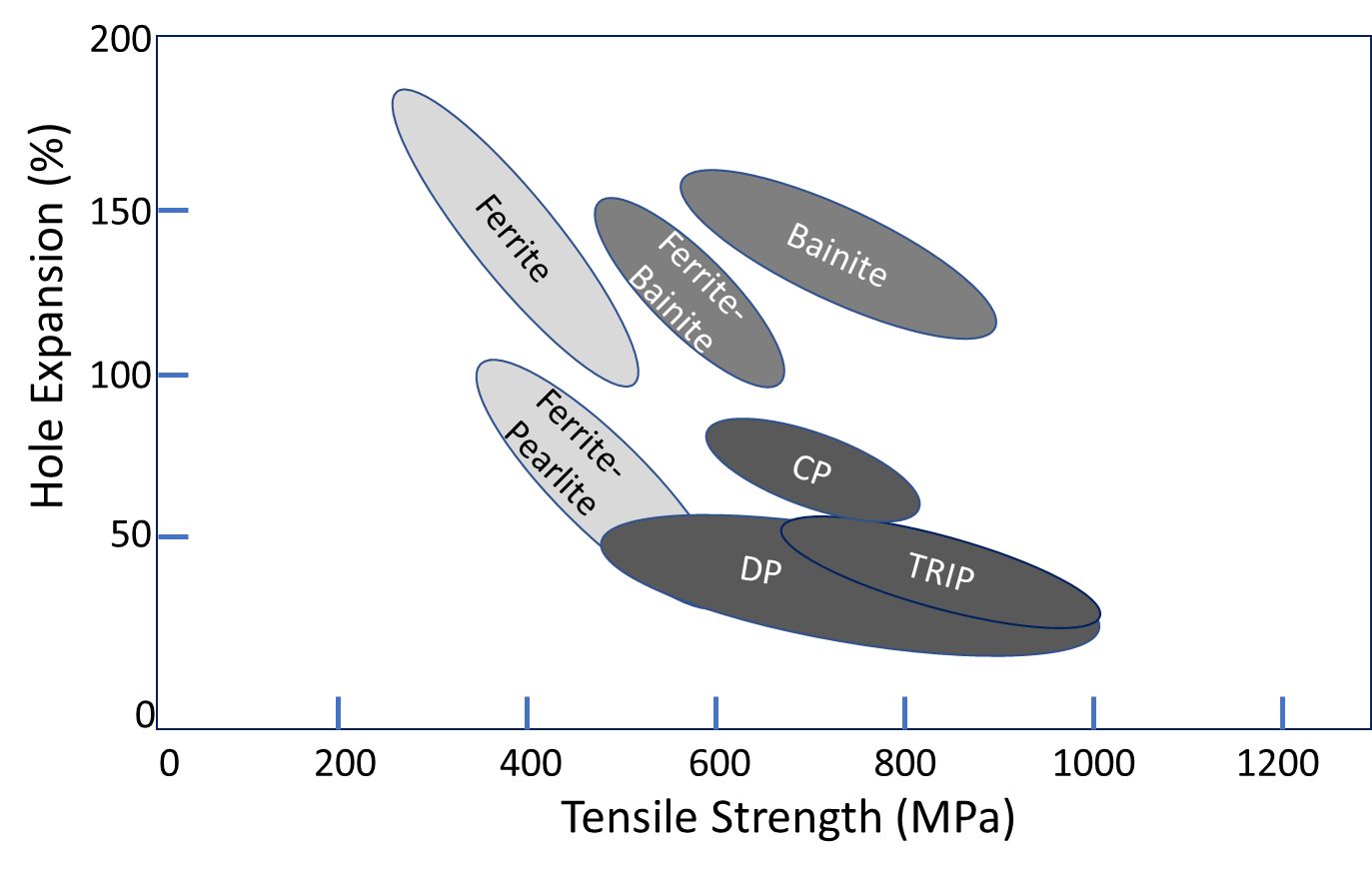
Figure 2: Hole Expansion as a Function of Strength and Microstructure. Adapted from Citation M-11.
An example of the impact of these modifications is shown in a paper published by C. Chiriac and D. HoydickC-10, where a 1 mm DP780 galvannealed steel was modified to produce a grade with improved hole expansion to achieve greater resistance to local formability failures such as edge fracture and shear fracture. These changes were made while retaining the same base metal chemistry and the same fraction of martensite in the structure, and resulted in similar tensile strength and total elongation but with a 50% increase in hole expansion (Table I and Figure 3). The key difference is a lower martensite hardness, and a smaller difference between the hardness of the martensite and ferrite. The modified DP grade has more homogeneous distribution of martensite with smaller ferrite and martensite grains (Figure 4).

Table I: Comparison of a conventional DP780 steel with a similar chemistry modified to improve hole expansion.C-10
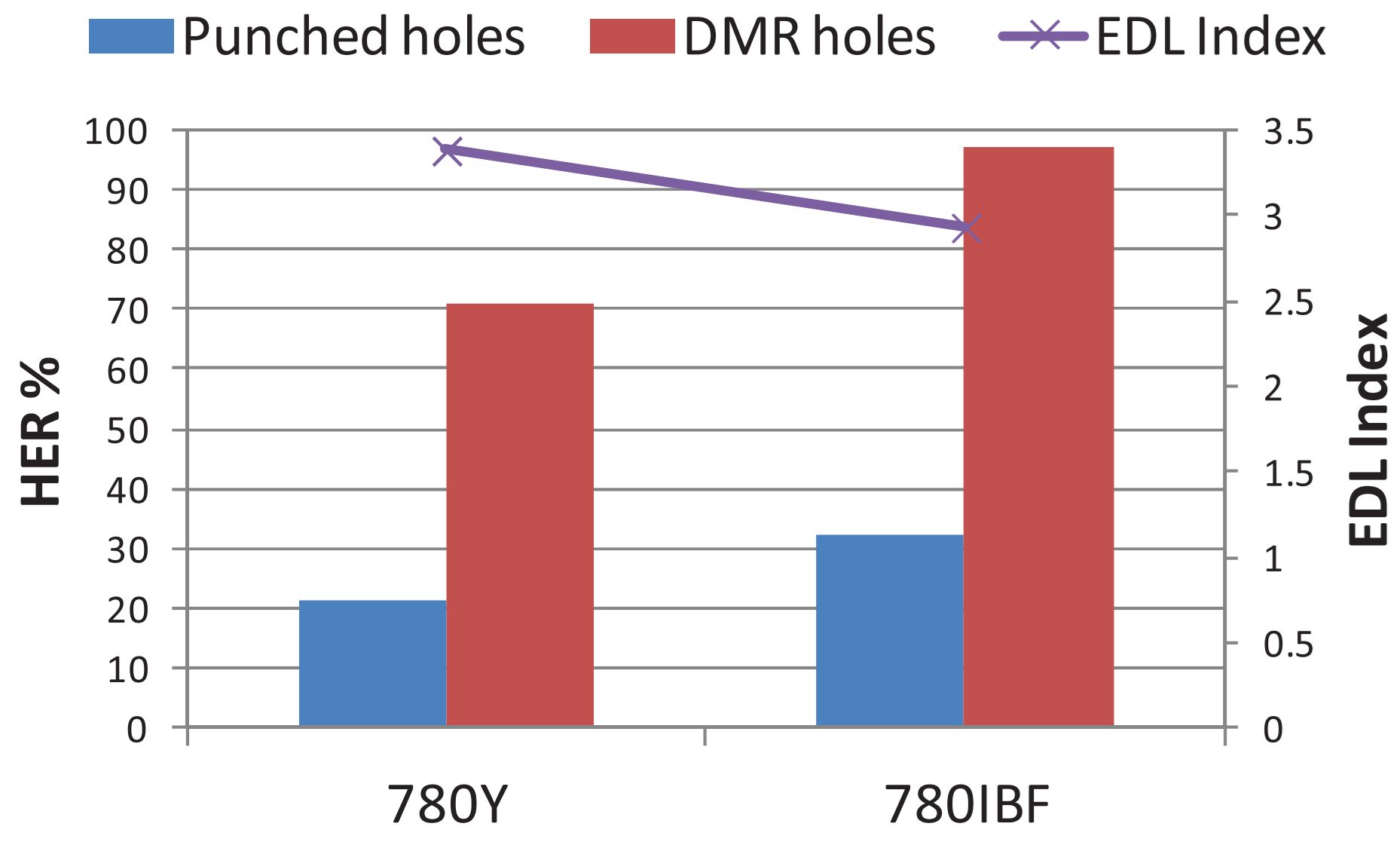
Figure 3: Improvement in Hole Expansion improves with grade modifications and edge quality. DMR = drilled, milled, and reamed hole; EDL = Edge Ductility Loss index, the ratio of the hole expansion of the DMR hole to that of the punched hole.C-10
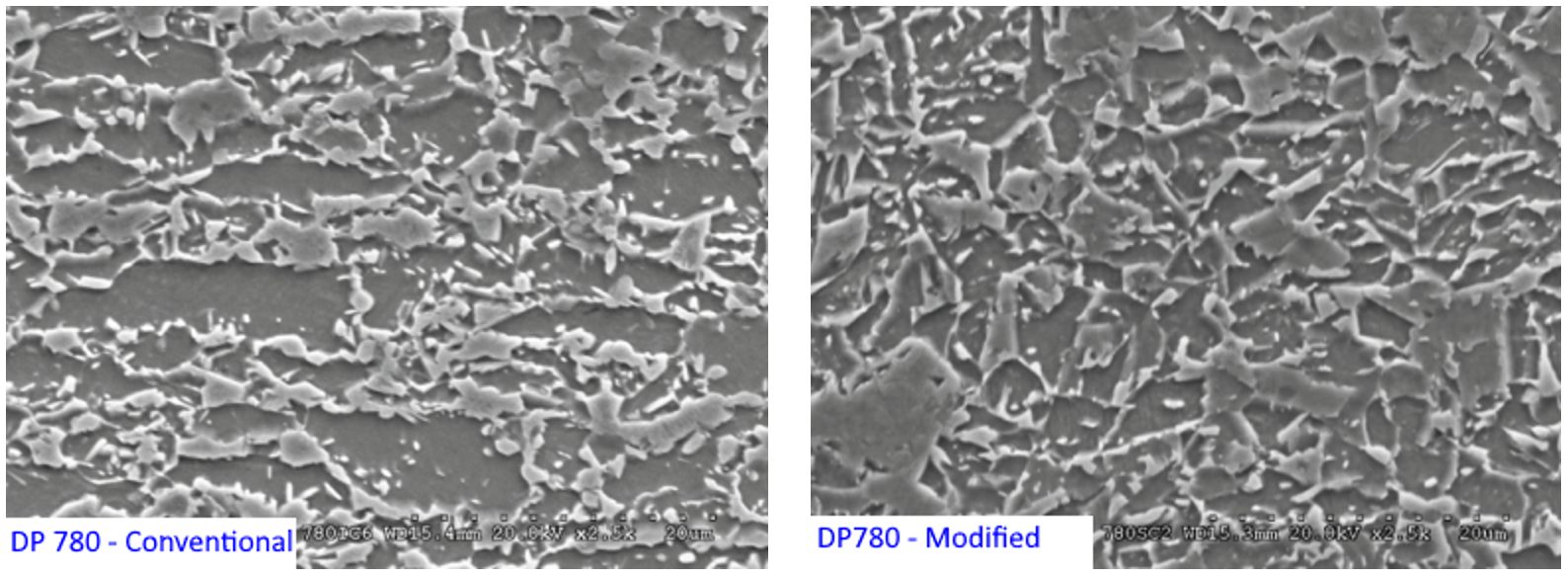
Figure 4: Comparison of the microstructure of a conventional DP780 steel (left) with a similar chemistry modified to improve hole expansion (right). Overall, there is the same fraction of martensite in both grades, but the modified chemistry has finer features.C-10
A presentation at a 2020 conferenceK-16 described a study which compared DP780 from six different global suppliers. Hole expansion tests were done on 1.4 mm to 1.5 mm mm thick samples prepared with either a sheared edge at 13% clearance, a sheared edge with 20% clearance, or a machined edge. Not surprisingly, the machined edge with minimal work hardening outperformed either of the sheared edge conditions. However, when considering only the machined edge samples, the hole expansion ratio ranged from below 30% to more than 70% (Figure 5). Presumably the only difference was the microstructural characteristics of the six DP780 products.

Figure 5: Variation in hole expansion performance from DP780 from 6 global suppliers.K-56
The microstructural differences that enhance local formability characteristics may be detrimental to global formability characteristics and vice versa. Conventional dual phase steels, with a soft ferrite matrix surrounding hard martensite islands, excel in applications where global formability is the limiting scenario. These steels have a low YS/TS ratio and high total elongation. However, the interface between the ferrite and martensite is the site of failures that limit the sheared edge extension of these grades. On the other end of the spectrum, fully martensitic grades are the highest strength steels available. These have a high YS/TS ratio, and low total elongation. Having only a single phase helps these grades achieve surprisingly high hole expansion values considering the strength, as seen in Figure 6.
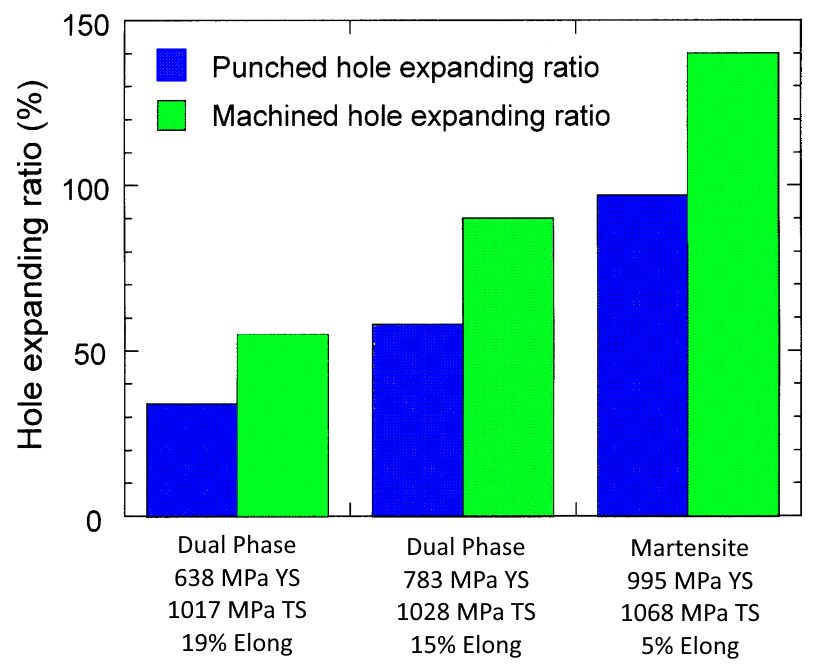
Figure 6: Hole Expansion as a Function of Edge Quality and Microstructure. Adapted from Citation.H-7
Knowing that a higher volume fraction of martensite is needed to increase strength, combined with the awareness that minimizing the hardness differences between microstructural phases is needed to increase hole expansion (Figure 7), allows steelmakers to fine-tune their chemistry and mill processing to target specific balances of strength, tensile elongation, and cut edge expandability as measured in a tensile test.
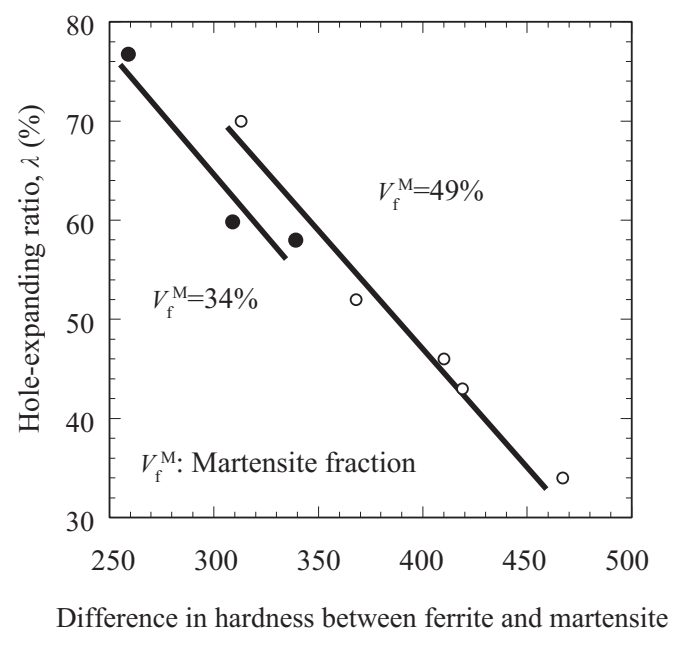
Figure 7: Improved Hole Expansion by Reducing the Hardness Difference between Ferrite and Martensite.H-8
This expands the selection of grades from which manufacturers can choose. Traditional material selection and identification may have been based on tensile strength to satisfy structural requirements – DP980 is a dual phase steel with 980MPa minimum tensile strength. However, newly engineered grade options offer users an extra level of refinement depending on the functional needs of the part. Products can be specified as needing high tensile elongation, high hole expansion, or a balance of these two. In the example shown in Figure 8, note that all 3 grades have nearly identical tensile strength.
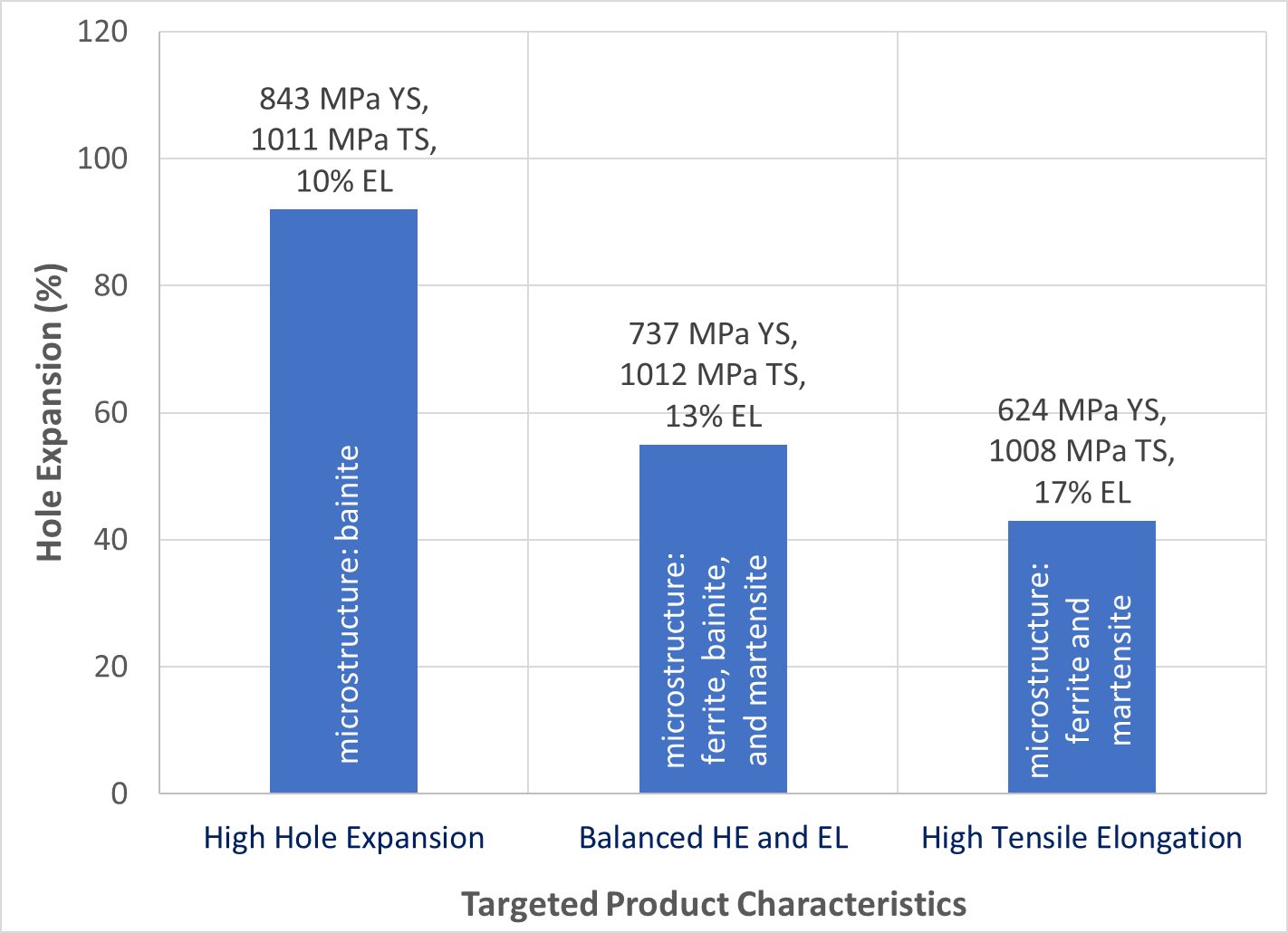
Figure 8: Engineered Microstructures Achieve Targeted Product Characteristics. (Data from Citations N-8 and F-5)
The influence of microstructure and the hardness differences between the phases is also seen in the hole expansion values of AHSS grades at strengths below 980 MPa. A study from 2016 shows the impact of a small amount of martensite on a ferrite-bainite microstructure.N-9 Both products compared had a microstructure of 80% ferrite. In one product, the remaining phase was only bainite, while the other had both martensite and bainite. The presence of just 8% martensite was sufficient to decrease the hole expansion capacity by 40%. (Figure 9).
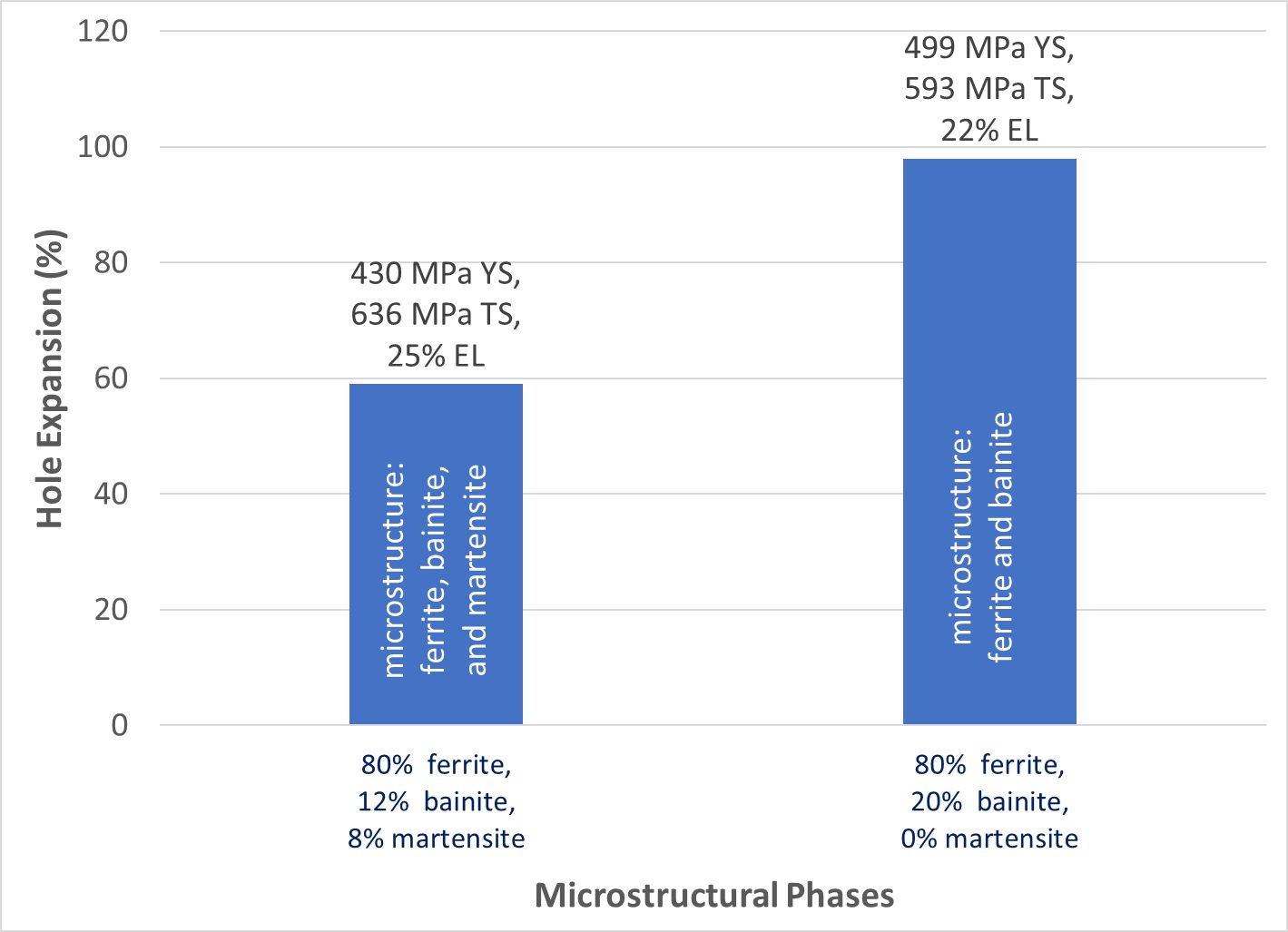
Figure 9: High Hardness Differences in Microstructural Phases Decrease Edge Ductility at All Strength Levels. Adapted from Citation N-9.
Rolling direction may also influence edge fracture sensitivity on some multiphase AHSS grades. When testing a sample, edge fractures may occur first at the hole edge along the rolling direction, which corresponds to a tensile axis in the transverse direction. If the chosen grade exhibits this behavior, locate stretch flanges perpendicular to the rolling direction when possible during die and process development to increase resistance to edge fracture. If this is not practical, identify locations where inserting scallops/notches in the stretch flange will not negatively impact the part structure, fit or die processing.
During die development and die try-out, it is important to use the production-intent AHSS grade – not just one that has the same tensile strength. Blank orientation relative to the rolling direction in these trials must also be production-intent. Often the blank die is the last completed die, so prototype blanks may be prepared by laser, EDM, water jet or even by hand during tryout. These cutting methods will have different sheared edge extension, as measured by the hole expansion test, compared with the production-intent shearing. These differences may be sufficiently significant to prevent replication of production conditions in tryout.
Blog, homepage-featured-top, main-blog
Bubble chart. Banana diagram. Steel strength ductility diagram—it’s been called a lot of things over the years. But the 2021 chart shown in Figure 1 is the subject of hundreds of requests for use we receive from engineers and students all over the world and appears in thousands of presentations and papers. Because of that, we periodically update it to make sure it reflects the most current picture of both commercially available, as well as emerging steel grades. In this blog, we are providing our updated GFD for download as well as definitions of steel classifications, as agreed to by our member companies.

Figure 1: The 2021 Global Formability Diagram comparing strength and elongation of current and emerging steel grades.
Steel Classifications
There are different ways to classify automotive steels. One is a metallurgical designation providing some process information. Common designations include lower-strength steels (interstitial-free and mild steels); conventional high strength steels, such as bake hardenable and high-strength, low-alloy steels (HSLA); and Advanced High-Strength Steels (AHSS) such as dual phase and transformation-induced plasticity steels. Additional higher strength steels include press hardening steels and steels designed for unique applications that have improved edge stretch and stretch bending characteristics.
A second classification method important to part designers is strength of the steel. This document will use the general terms HSLA and AHSS to designate all higher strength steels. The principal difference between conventional HSLA steels and AHSS is their microstructure. Conventional HSLA steels are single-phase ferritic steels with a potential for some pearlite in C-Mn steels.
AHSS are primarily steels with a multiphase microstructure containing one or more phases other than ferrite, pearlite, or cementite – for example martensite, bainite, austenite, and/or retained austenite in quantities sufficient to produce unique mechanical properties. Some types of AHSS have a higher strain hardening capacity resulting in a strength-ductility balance superior to conventional steels. Other types have ultra-high yield and tensile strengths and show a bake hardening behavior.
What are 3rd Gen Steels?
Third Generation, or 3rd Gen, AHSS builds on the previously developed 1st Gen AHSS (DP, TRIP, CP, MS, and PHS) and 2nd Gen AHSS (TWIP), with global commercialization starting around 2020. Third Gen AHSS are multi-phase steels engineered to develop enhanced formability as measured in tensile, sheared edge, and/or bending tests. Typically, these steels rely on retained austenite in a bainite or martensite matrix and potentially some amount of ferrite and/or precipitates, all in specific proportions and distributions, to develop these enhanced properties.
Graphical Presentation
Generally, elongation (a measure of ductility) decreases as strength increases. Plotting elongation on the vertical axis and strength on the horizontal axis leads to a graph starting in the upper left (high elongation, lower strength) and progressing to the lower right (lower elongation, higher strength). When considering conventional steels and the first generation of advanced high strength steels, this shape led to the colloquial description of calling this the banana diagram.
With the continued development of advanced steel options, it is no longer appropriate to describe the plethora of options as being in the shape of a banana. Instead, with new grades filling the upper right portion of Figure 1, perhaps it is more accurate to describe this as the football diagram as the options now start to fall into the shape of an American or Rugby Football. Officially, it is known as the steel Global Formability Diagram.
Even this approach has its limitations. Elongation is only one measure of ductility. Other ductility parameters are increasingly important with AHSS grades, such as hole expansion and bendability. There are several other approaches that have been proposed by experts around the world. Have a look at our Defining Steels article, from which this article was drawn, to learn more about them. You will also find within Defining Steels a detailed explanation of the nomenclature used throughout the Guidelines to define steels. If you have questions, please use the Comments tool below or on the Defining Steels page.
Download the GFD
Because of its popularity, we provide high resolution image files of the GFD here for your download and use. Please source it “Courtesy of WorldAutoSteel” in your papers and presentations. We are happy for you to use it. If you require our signed permission, please write us at steel@worldautosteel.org. We’ll respond quickly.
* The Guidelines use the general terms HSLA and AHSS to designate all higher strength steels.
Citations
Citation:
M-66. M. Mazar Atabaki, J. Ma, W. Liu, R. Kovacevic, “Pore formation and its mitigation during hybrid laser/arc welding of advanced high strength steel”, Materials & Design, Volume 67, 2015, Pages 509-521, ISSN 0261-3069, https://doi.org/10.1016/j.matdes.2014.10.072.
Arc Welding
This is a summary of a paper of the same title, authored by K. Májlinger, E. Kalácska, and P. Russo Spena, used by permission.M-65
Researchers at the Budapest University of Technology and Economics and the Free University of Bozen-Bolzano tested gas metal arc welding (GMAW) of dissimilar Advanced High-Strength Steel (AHSS) sheets.M-65 The test pieces were 100 x 50 mm samples of 1.4 mm TWIP (TWIP1000) and 0.9 mm TRIP (HCTC800T) sheet steels were welded in a lap joint configuration with 0.8 mm diameter AWS ER307Si austenitic stainless steel wire to determine appropriate GMAW parameters for good quality welds. Quality was determined by external appearance, microstructure, and mechanical properties. Good welds were achieved with linear heat inputs (Q) with ranges from 500-650 kJ/m. The only fractures that occurred appeared within the weld bead by ductile failure modes. The HAZ of the TWIP steel showed grain coarsening and the HAZ of the TRIP steel experienced microstructural changes relative to the distance from the fusion boundary. The ultimate tensile strength (UTS) varies between 73%-84% of the weaker of the two steels.
Welding was conducted with an automated linear drive system with pure Argon (99.996% Ar) shielding gas at 10L/min. Wire feed rates were approximately 3.5 m/min with Direct Current Electrode Positive (DCEP) polarity. Changes in current, voltage, weld speed, and the resulting linear energy are compared in Table 1.
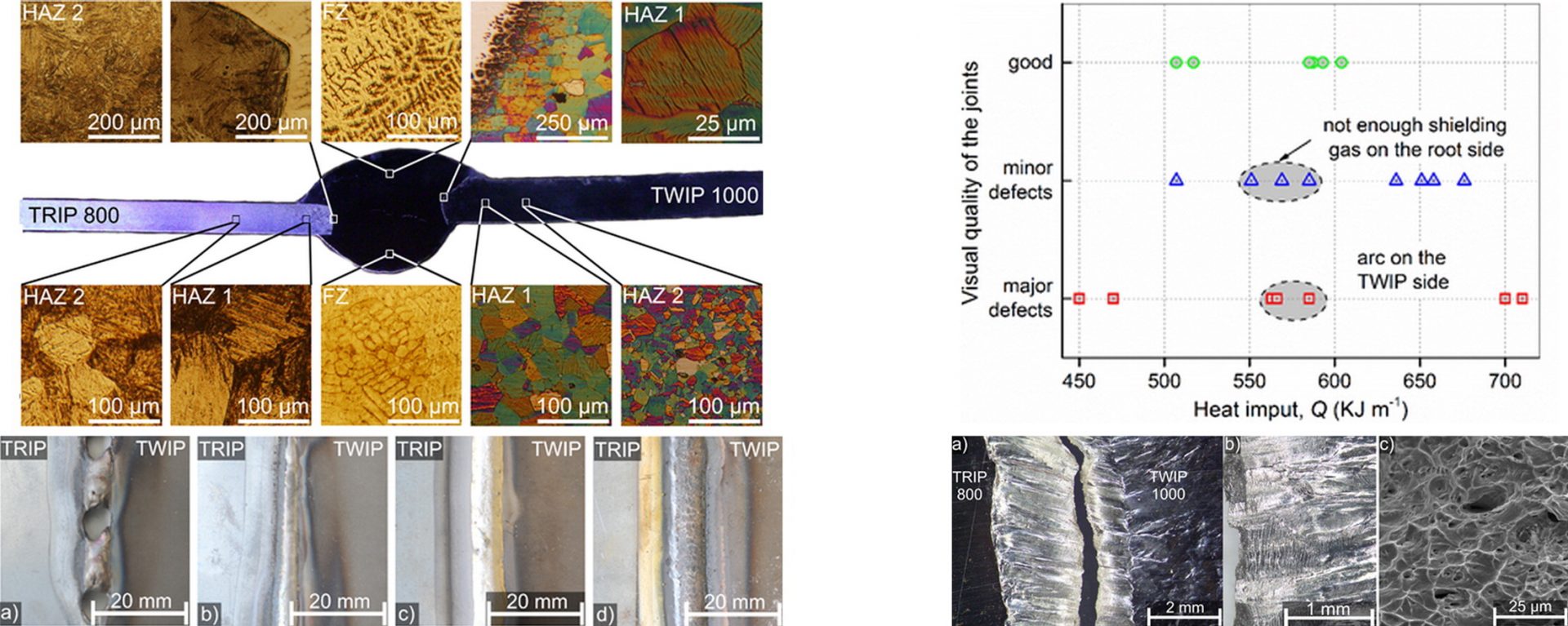
Figure 1: Overview of Dissimilar AHSS GMAW Welding.M-65
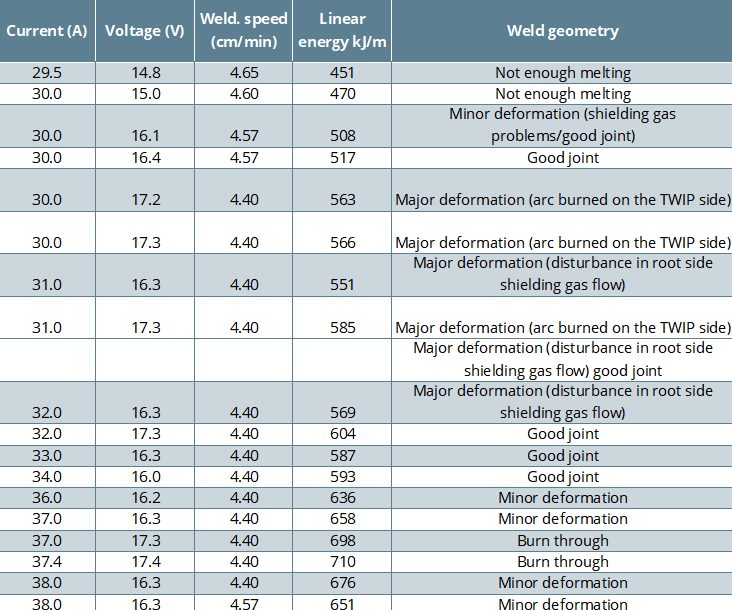
Table 1: Results of the preliminary welding tests in terms of TWIP-TRIP joint quality.M-65
After welding, transverse sections were cut from the welds and etched to show the microstructure. Vickers hardness testing was conducted on the weld samples based on the ASTM E384 standard. Tensile tests were performed on the samples according to the EN ISO 6892-1 standard. Tests were also conducted on unwelded TWIP and TRIP steels for comparison. Scanning electron microscopy (SEM) examinations were made of fracture surfaces to determine failure modes and examine for microscopic weld defects.
The study concluded that dissimilar welds between AHSS steels with the GMAW process can be achieved with consistent results desired for automotive applications.
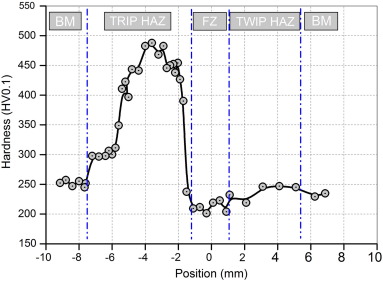
Figure 2: Vickers Hardness Across Weldment.M-65
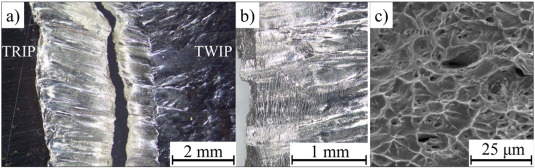
Figure 3: Ductile Failure in the fragile zones (FZ).M-65