1stGen AHSS, AHSS, Steel Grades
Ferrite-Bainite (FB) steels are hot rolled steels typically found in applications requiring improved edge stretch capability, balancing strength and formability. The microstructure of FB steels contains the phases ferrite and bainite. High elongation is associated with ferrite, and bainite is associated with good edge stretchability. A fine grain size with a minimized hardness differences between the phases further enhance hole expansion performance. These microstructural characteristics also leads to improved fatigue strength relative to the tensile strength.
FB steels have a fine microstructure of ferrite and bainite. Strengthening comes from by both grain refinement and second phase hardening with bainite. Relatively low hardness differences within a fine microstructure promotes good Stretch Flangable (SF) and high hole expansion (HHE) performance, both measures of local formability. Figure 1 shows a schematic Ferrite-Bainite steel microstructure, with a micrograph of FB 400Y540T shown in Figure 2.

Figure 1: Schematic Ferrite-Bainite steel microstructure.

Figure 2: Micrograph of Ferrite-Bainite steel, HR400Y540T-FB.H-21
The primary advantage of FB steels over HSLA and DP steels is the improved stretchability of sheared edges as measured by the hole expansion test. Compared to HSLA steels with the same level of strength, FB steels also have a higher strain hardening exponent (n-value) and increased total elongation. Figure 3 compares FB 450/600 with HSLA 350/450 steel. Engineering and true stress-strain curves for FB steel grades are shown in Figure 4.

Figure 3: A comparison of stress strain curves for mild steel, HSLA 350/450, and FB 450/600.
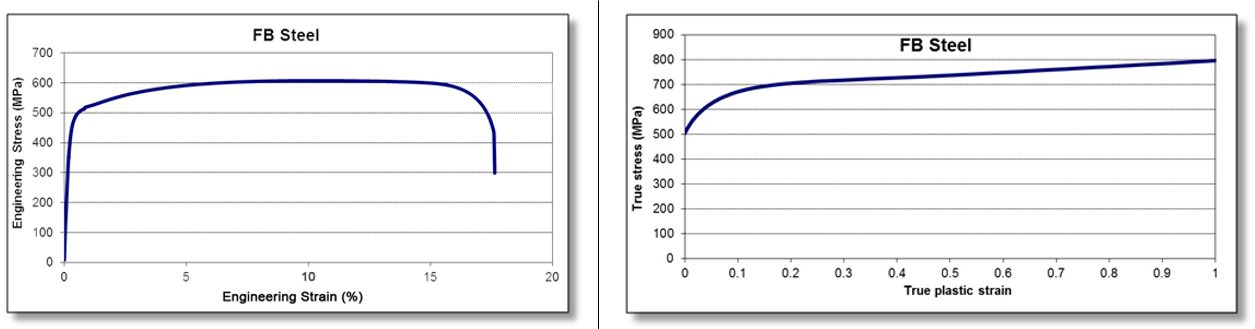
Figure 4: Engineering stress-strain (left graphic) and true stress-strain (right graphic) curve for FB 450/600.T-10
Examples of typical automotive applications benefitting from these high strength highly formable grades include automotive chassis and suspension parts such as upper and lower control arms, longitudinal beams, seat cross members, rear twist beams, engine sub-frames and wheels.
Some of the specifications describing uncoated hot rolled 1st Generation ferrite-bainite (FB) steel are included below, with the grades typically listed in order of increasing minimum tensile strength and ductility. Different specifications may exist which describe uncoated or coated versions of these grades. Many automakers have proprietary specifications which encompass their requirements.
- EN 10338, with the terms HDT450F and HDT580F D-18
- VDA239-100, with the terms HR300Y450T-FB, HR440Y580T-FB, and HR600Y780T-FB V-3
- JFS A2001, with the terms JSC440A and JSC590AJ-23
Lower Strength Steels, Steel Grades
Metallurgy of Ultra-Low Carbon Steels (DDS – EDDS)
ULC, IF, VD-IF, and EDDS are interchangeable terms that describe the most formable (high n-value) and lowest strength grade of steel.
Ultra-low-carbon (ULC) steels typically carbon levels less than 0.005%, or 50 parts per million. At these low alloying levels, the atomic structure is primarily iron, with unfilled spaces or gaps (called interstices) between the atoms – the origin of the term “interstitial-free” or IF. Molten steel needs an additional process prior to casting called vacuum degassing (VD) to reach these carbon levels. Because this steel alloy is mainly iron and all pure elements are very formable, it is also referred to as either deep drawing steel (DDS) or extra-deep-drawing steel (EDDS). Specifications which contain ULC grades are listed within the Mild Steels page.
Adding phosphorus to an IF grade increases the strength due to solid solution strengthening, precipitation of carbides and/or nitrides, and grain refinement. These higher strength IF-HS grades are widely used for both structural and closure applications. Work hardening from forming will increase panel strength, and is sometimes called a dent resistant steel grade. However, this alloying approach is not capable of producing a bake hardenable grade.
Lower Strength Steels, Steel Grades
Metallurgy of Mild Steels
Mild steels are low carbon steels with no alloying elements added for substantial strengthening, and for that reason are characterized by relatively lower yield strength. Typically, mild steels have less than 0.10% carbon.
These steels have a microstructure that is primarily ferrite. The amount of pearlite in the microstructure is a function of the amount of carbon in the steel, with lower carbon resulting in a lower fraction of pearlite. More information about microstructural components is available here.
Ultra-low carbon steels are a type of mild steel. These grades are typically the lowest yield strength and highest ductility available. Generally, these steels have less than 0.005% carbon, or less than 50 ppm C. More information is contained on the page for Ultra-Low Carbon steels.
Different names may describe mild steels, but these differences came from steel mill production techniques that are no longer in use. Since all sheet steels in use today are continuously cast, there are no significant differences between these terms:
- Drawing Quality (DQ)
- Drawing Steel (DS)
- Aluminum Killed Drawing Quality (AKDQ)
- Drawing Quality Aluminum Killed (DQAK)
- Drawing Quality Special Killed (DQSK)
Mild steels are described in OEM, regional or global specifications with different syntax. While certain grade definitions between these specifications may be similar, the user is cautioned against using conversion charts without first confirming aspects which might be different, such as minimum, maximum, or typical values of chemical or mechanical properties. For example, ASTM specifications which cover lower strength steels list only typical values for tensile properties, but these are non-mandatory and the user may receive product outside the ranges shown. Additionally, JIS specifications which cover lower strength steels do not have minimum yield strength requirements, and the minimum elongation varies by thickness.
Some of the specifications describing uncoated cold rolled mild steel are included below, with the grades typically listed in order of increasing ductility. Different specifications may exist which describe hot or cold rolled, uncoated or coated, or steels of different strengths. Many automakers have proprietary specifications which encompass their requirements.
- ASTM A1008M, with the terms CS, DS, DDS, and EDDSA-25
- EN10130, with the terms DC01, DC03, DC04, DC05, and DC06D-3
- JIS G3141, with the terms SPCC, SPCD, SPCE, SPCF, and SPCGJ-2
- JFS A2001, with the terms JSC270C, JSC270D, JSC270E, JSC270F, and JSC260GJ-23
- VDA239-100, with the terms CR1, CR2, CR3, CR4, and CR5V-3
Forming
Introduction
Approaches for forming higher strength steels evolved with the commercialization of increased strength levels of High Strength Low Alloy (HSLA) steels. Demands for greater crash performance while simultaneously reducing mass and cost have spawned the development of new groups of steels that improve on the properties of these HSLA steels. Forming of Advanced High-Strength Steel (AHSS) is not a radical change from forming conventional HSLA steels, providing some of the key differences are understood and accounted for in die design, die process, and equipment selection.
AHSS grades solve two distinct automotive needs by two different groups of steels. The first group as a class has higher strength levels with improved formability and crash-energy absorption compared to HSLA grades. DP, TRIP, FB, and TWIP steels, which have increased values of the work hardening exponent (n-value), fulfill this requirement. The second group, including CP and MS steels, extends the availability of steel in strength ranges above what is available with HSLA grades. Originally targeted for chassis, suspension, and body-in-white components, AHSS grades are now being applied to doors and other body panels. New variations in microstructure help meet specific process requirements, including increased edge stretch, bendability, strengthening after forming, or tighter property tolerances.
The progressive increases in yield and tensile strength with these new AHSS grades magnifies existing forming issues with conventional HSLA grades and creates new challenges. Concerns include higher loads on processing equipment including presses, levelers, straighteners, blanking lines, coil slitting lines and roll forming equipment. Additionally, there are material and surface treatment considerations required for tooling in the stamping plants: draw dies, trim steels, and flange steels. Compared to conventional HSLA steels, greater energy requirements result from higher AHSS yield strengths, tensile strengths and significantly higher work hardening rates. This places new requirements on press capacity, leveler, straightener and slitting capabilities, tool construction/protection, lubricant capabilities, part and process design, and maintenance. Springback management becomes more critical as yield strengths continue to increase. Conventional and press hardened (hot formed) AHSS parts have very high strength after forming, so re- forming operations should be avoided. Trimming, cutting, and piercing equipment must be constructed and maintained to overcome the extreme high strength of the final stamping. Laser cutting of press hardened parts produces a finished part that avoids pushing the limits of trim and pierce tools and dies utilized for conventional HSLA steel.
There are an ever-increasing number of AHSS multiphase microstructure grades available, each designed to resist various forming failure modes while achieving final part performance requirements. Sharing of information regarding the planned part geometry, die and stamping processing, and final part application between steel suppliers, product and die process engineering, and end users helps ensure selection of the right steel grade for the application. This becomes especially relevant since multiphase microstructures experience additional forming failure modes compared with conventional high strength products.
Tool Design Considerations
The characteristics associated with different AHSS grades influence die design and die processing decisions. Not only are these steels typically higher in strength, but they also undergo substantial work hardening during forming. These lead to increased local loads, and changes in friction, die wear, and press requirements. The multiphase microstructures increase cut edge and bending fracture sensitivity. As such, extending the life and performance of tooling in press shops requires a rethinking of tool and part design.
Part Design
Successful application of any material requires close coordination of part design and the manufacturing process. Consult product and manufacturing process engineers when designing AHSS parts to understand both the limitations and advantages of the grade and the proper forming process to be employed. Start in the concept and feasibility stage to ensure sufficient time for corrective actions and optimization.
Soft tool materials like kirksite may be used for manufacturing prototype parts and the inserts used to eliminate local wrinkles or buckles. However, wear resistant coatings are typically not applied to these tool surfaces, so the metal flow seen in these prototype parts may not match the metal flow seen under production conditions. The results from soft tool tryouts should not be used to assess manufacturability and springback of AHSS parts.
Design structural frames (such as rails, sills, cross members, and roof bows) as open-ended channels to permit forming operations rather than draw die processes. AHSS stampings requiring closed-end draw operations are limited by a reduced depth of draw, Figure 1. Less complex, open-ended stamped channels are less limited in depth. A rule of thumb is that DP 350Y600T can be formed to only half the draw depth of a mild steel.

Figure 1: Schematic of an open end part design (left) and a closed end part design (right). The open-ended design allows for greater depths when utilizing AHSS versus the closed ended design historically used with mild steel.A-5
Where possible, avoid closed-end developments to make more complex geometries with AHSS grades. Wrapping ends of “hat” sections increases forming loads, increases the chances of circumferential compression wrinkling on the binder, specifically in the corners, and increases wrinkling on the draw wall if the blank edge runs through the draw bead. Draw die developments that include a closed (or wrapped) end development usually also require a larger blank size. During draw die development, it is best to identify parts that have a “hat” section geometry in certain locations and develop the draw die accordingly to maximize the positive formability attributes of AHSS while minimizing the limitations of AHSS.
For example, the left image in Figure 2 shows a draw die development on a DP600 cowl side with a closed (wrapped) end, with the right image showing a similar part developed with an open end. Although both final part geometries are similar, the closed-end development led to significant global formability failures due to the excessive stretch. In contrast, the open-ended development had virtually no global formability related failures. Other design and die development differences in the part on the right include the use of stake beads to control springback and embossments to eliminate wavy metal. In addition, an open-ended development has the potential to reduce the blank size for material utilization savings.

Figure 2: Draw die development for a cowl side formed from DP600. Left image: closed-end development with global formability failures, waviness, and springback. Right image: open-ended development with no splits, waves, or dimensional concerns.U-6
The automotive industry has adopted a strategy for “lighter dies and fewer dies”, to reduce cost. One key element is “part consolidation”, such as one-piece body side outers and inners. High strength steels challenge the part consolidation mantra. When encountering extreme formability challenges, parts previously made with one set of dies when stamped from lower strength steels may benefit from transitioning to a laser welded blank with a lower strength grade in the challenging region and higher strength steels in the remainder of the part. Alternatively, splitting the consolidated part into two or more separate parts subsequently welded together may improve stamping success at the expense of another operation. In the past, one-piece rocker panels were stamped from conventional mild or HSLA steel. However, this component requires higher strength and reduced thickness to meet weight and crash requirements, so now DP980 is often considered as the grade of choice for this application. Figure 3 shows a rocker panel where insufficient formability of DP980 prevented a one-piece stamping. The OEM solved this by dividing the part into two stampings, putting a more formable grade where needed on the wrapped (or closed) end.

Figure 3: When a one-piece rocker panel could not be successfully formed from DP980, the OEM stamped a DP980 rocker panel section with an open-ended design and spot welded it to a mild steel end cap.U-6
Trim and Pierce Tool Design
- Trim and pierce tools need to withstand higher loads since AHSS grades have higher tensile strengths than conventional high-strength steels.
- Edge cracking is minimized with proper support of the trim stock during trimming.
- Modify timing of the trim/pierce operation to minimize snap-through reverse loading.
- Scrap shedding may be an issue, since AHSS springback can cause scrap to stick in the tool.
Flange Design
- Design more formable flanges to reduce need for extra re-strike operations.
- Areas to be flanged should have a “break-line” or initial bend radius drawn in the first die to reduce springback.
- Adapt die radii for material strength and blank thickness.
Draw Bead Design
- Metal flow across draw beads generates strain and minimizes the elastic recovery which causes springback.
- Metal flow across draw beads generates large amounts of work hardening, leading to increased press loads.
- Optimizing blank size and shape reduces the reliance on draw beads, which can excessively work harden the material before entering the die opening.
Guidelines to Avoid Edge Cracking During Stretch Flanging
- Flange length transition should be gradual – abrupt changes in flange length cause local stress raisers leading to edge cracks.
- Use good cutting practices to achieve a high-quality edge.
- Avoid the use of sharp notch features in curved flanges.
- Avoid putting bypass notches in stretch or compression edges of blanks or progressive die carrier strips. These bypass notches can act as stress risers and lead to edge fractures in the draw or flange operation. In addition, bypass notches in blanks and progressive dies are difficult to maintain, which can increase the potential for edge fracture.
- Metal gainers in the draw die or in the die prior to the stretch flange operation compensates for change in length of line that occurs during flanging, helping to avoid edge cracking. In the example shown in Figure 4, edge fractures moved from the draw panel to flanged panel after grinding on the draw die to eliminate edge fractures in the draw operation. The draw panel underneath the flanged part in Figure 4 did not have edge fractures. The reduction in the length of line in the draw operation moved the problem to the flanged part where the stamping transitioned from bending and straightening in the flange operation to a stretch flange operation. A better practice is to add metal gainers to the draw panel to provide the feedstock which expands during stretch flanging.

Figure 4: Flanged panel fractures, with the draw panel underneath. Adding metal gainers to the draw panel would help minimize these fractures.U-6
- The higher strength of AHSS makes it more difficult to pull out loose metal or achieve a minimum stretch in flat sections of stampings. Addendum, metal gainers (Figures 5 and 6), and other tool features balance lengths of line and locally increase stretch.

Figure 5: Metal gainers help avoid insufficient stretched areas and eliminate buckles.T-3

Figure 6: Metal gainers and depressions balance stresses and minimizes wrinkled metal.A-41
2ndGen AHSS, AHSS, Steel Grades
TWinning Induced Plasticity (TWIP) steels have the highest strength-ductility combination of any steel used in automotive applications, with tensile strength typically exceeding 1000 MPa and elongation typically greater than 50%.
TWIP steels are alloyed with 12% to 30% manganese that causes the steel to be fully austenitic even at room temperature. Other common alloying additions include up to 3% silicon, up to 3% aluminum, and up to 1% carbon. Secondary alloying additions include chromium, copper, nitrogen, niobium, titanium, and/or vanadium.D-29 The high alloying levels and substantially greater levels of strength and ductility place these into the 2nd Generation of Advanced High Strength Steels. Furthermore, due to the density of the major alloying additions relative to iron, TWIP steels have a density which is about 5% lower than most other steels.
Calling this type of steel TWIP originates from the characteristic deformation mode known as twinning. Deformation twins produced during sheet forming leads to microstructural refinement and high values of the instantaneous hardening rate (n-value). The resultant twin boundaries act like grain boundaries and strengthen the steel. On either side of a twin boundary, atoms are located in mirror image positions as indicated in the schematic microstructure shown in Figure 1. Figure 2 highlights the microstructure of TWIP steel after annealing and after deformation.

Figure 1: Schematic of TWIP steel microstructure.

Figure 2: TWIP steel in the annealed condition (left) and after deformation (right) showing deformation twins. The number of deformation twins increases with increasing strain.K-42
EDDS or Interstitial-Free or Ultra-Low Carbon steels are different descriptions for the most formable lower-strength steel. Possible test results for this grade are 150 MPa yield strength, 300 MPa tensile strength, 22% to 25% uniform elongation, and 45% to 50% total elongation. In contrast, test results on TWIP steels may show 500 MPa yield strength, 1000 MPa tensile strength, 55% uniform elongation, and 60% total elongation.
The stress-strain curves for these two grades are compared in Figure 3. The TWIP curves show the manifestation of Dynamic Strain Aging (DSA), also known as the PLC effect, with more details to follow.

Figure 3: Uniaxial tensile stress-strain curves for an interstitial-free (IF) extra-deep-drawing steel and an austenitic Fe-18%Mn-0.6%C-1.5%Al TWIP steel. Curves are presented both terms of engineering (s,e) and true (σ,ε) stresses and strains, respectively.D-30
Figure 4 compares the results of bulge testing ferritic interstitial-free (IF) steel and austenitic Fe-18%Mn-0.6%C-1.5%Al TWIP steel. The TWIP steel is still undamaged at a dome height that is 31% larger than the IF steel dome height at failure.D-30

Figure 4: Comparison of dome testing between EDDS and TWIP.D-30
Excellent stretch formability is associated with high n-values. Shown in Figure 5 is a plot showing how the instantaneous n-value changes with applied strain. N-value increases to a value of 0.45 at an approximate true (logarithmic) strain of 0.2 and then remains relatively constant until an approximate true strain of 0.3 before increasing again. The high and uniform n-value delays necking and minimizes strain peaks. Twins continue to form at higher strains, leading to finer microstructural features and continued increases in n-value at higher strains.

Figure 5: Instantaneous n-value changes with applied strain. TWIP steels have high and uniform n-value leading to excellent stretch formability.C-30
A microstructural deformation phenomenon known as the Portevin-LeChatelier (PLC) effect occurs when deforming some TWIP steels to higher strain levels. The PLC effect is known by several other names as well, including jerky flow, discontinuous yielding, and dynamic strain aging (DSA).
The severity varies with alloy, strain rate, and deformation temperature. Figure 6 shows how DSA affects the appearance of the stress strain curve of two TWIP alloys.D-29 The primary difference in the alloy design is the curves on the right are for steel containing 1.5% aluminum, with the curves on the left for a steel without aluminum. The addition of aluminum delays the serrated flow until higher levels of strain. Note that both alloys have negative strain rate sensitivity.

Figure 6: Influence of aluminum additions on serrated flow in Fe-18%Mn-0.6%C TWIP (Al-free on the left) and Fe-18%Mn-0.6%C-1.5% Al TWIP (Al-added on the right).D-29
The primary macroscopic manifestations of the Portevin-LeChatelier (PLC) effect areD-29:
- negative strain rate sensitivity.
- stress-strain curve showing serrated or jerky flow, indicating non-uniform deformation. Strain localization takes place in propagating or static deformation bands.
- the strain rate within a localized band is typically one order of magnitude larger, while that outside the band is one order of magnitude lower, than the applied strain rate.
- limited post-uniform elongation, meaning uniform elongation is just below total elongation. Said another way, fracture occurs soon after necking initiation.
The PLC effect leads to relatively poor sheared edge expansion, as measured in a hole expansion test. Figure 7 on the left highlights the crack initiation site in a sample of highly formable EDDS-IF steel, showing the classic necking appearance with extensive thinning prior to fracture. In contrast, note the absence of necking in the TWIP steel shown in the right image in Figure 7.D-29
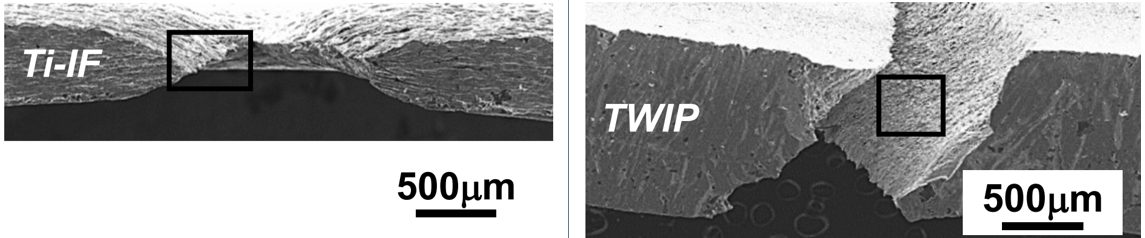
Figure 7: Sheared edge ductility comparison between IF (left) and TWIP (right) steel. TWIP steels lack the sheared edge expansion capability of IF steels.D-29
The stress-strain curves of several TWIP grades are compared in Figure 8.

Figure 8: Engineering stress-strain curve for several TWIP Grades.P-18
Complex-shaped parts requiring energy absorption capability are among the candidates for TWIP steel application, Figure 9.

Figure 9: Potential TWIP Steel Applications.N-24
Early automotive applications included the bumper beam of the 2011 Fiat Nuova Panda (Figure 10), resulting in a 28% weight savings and 22% cost savingsN-24 over the prior model which used a combination of PHS and DP steels.D-31

Figure 10: Transitioning to a TWIP Bumper Beam Resulted in Weight and Cost Savings in the 2011 Fiat Nuova Panda. N-24, D-31
In the 2014 Jeep Renegade BU/520, a welded blank combination of 1.3 mm and 1.8 mm TWIP 450/950 (Figure 11) replaced a two-piece aluminum component, aiding front end stability while reducing weight in a vehicle marketed for off-road applications.D-31

Figure 11: A TWIP welded blank improved performance and lowered weight in the 2014 Jeep Renegade BU/520.D-31
Also in 2014, the Renault EOLAB concept car where the A-Pillar Lower and the Sill Side Outer were stamped from TWIP 980 steel.R-21 By 2014, GM Daewoo used TWIP grades for A-Pillar Lowers and Front Side Members, and Hyundai used TWIP steel in 16 underbody parts. Ssangyong and Renault Samsung Motors used TWIP for Rear Side Members.I-20
Other applications include shock absorber housings, floor cross-members, wheel disks and rims, wheelhouses, and door impact beams.
A consortium called TWIP4EU with members from steel producers, steel users, research centers, and simulation companies had the goal of developing a simulation framework to accurately model the complex deformation and forming behavior of TWIP steels. The targeted part prototype component was a backrest side member of a front seat, Figure 12. Results were published in 2015.H-58

Figure 12: TWIP4EU Prototype Component formed from TWIP Steel.H-58
In addition to a complex thermomechanical mill processing requirements and high alloying costs, producing TWIP grades is more complex than conventional grades. Contributing to the challenges of TWIP production is that steelmaking practices need to be adjusted to account for the types and amounts of alloying. For example, the typical ferromanganese grade used in the production of other grades has phosphorus levels detrimental to TWIP properties. In addition, high levels of manganese and aluminum may lead to forming MnO and Al2O3 oxides on the surface after annealing, which could influence zinc coating adhesion in a hot dip galvanizing line.D-29